The end goal of our energy systems is to cause energy exchanges that allow us to do useful work.
In Part II, we saw that all the useful work we require can be reduced to the 4 primary energy needs: heating, lighting, movement, and computation.
You may have noticed that the technologies we use to serve these needs only take energy input in 2 forms: heat and electricity.
Energy production describes the set of methods we use to generate heat and electricity.
In this section, we'll look into all the most important production methods and understand how they capture energy from our environment.
Then, we'll evaluate which production methods are best suited to meet our growing long-term energy demands.
Many of the demonstrative calculations and explanations in this section are distilled from Tom Murphy's incredible textbook Energy and Human Ambition on a Finite Planet, which I would highly recommend to anyone curious to go deeper into understanding energy production.
Fossil Fuels
Coal
Coal was the first major fossil fuel energy source. The discovery of coal and invention of the steam engine was arguably the cause of the Industrial Revolution.
Coal is the cheapest and most abundant fossil fuel. Today, it is the largest source of electricity, making up ~37% of electricity production in 2022
Coal has a high concentration of carbon. This carbon can react with oxygen in a combustion reaction to produce carbon-dioxide and a net energy output released as heat.
coal combustion reaction
Coal can be burned in power plants to heat up water and produce steam. This steam then creates pressure to spin a turbine, generating electricity. This the standard pattern for how power plants generate heat. We'll learn how the spinning turbine generates electricity in detail in Part IV.
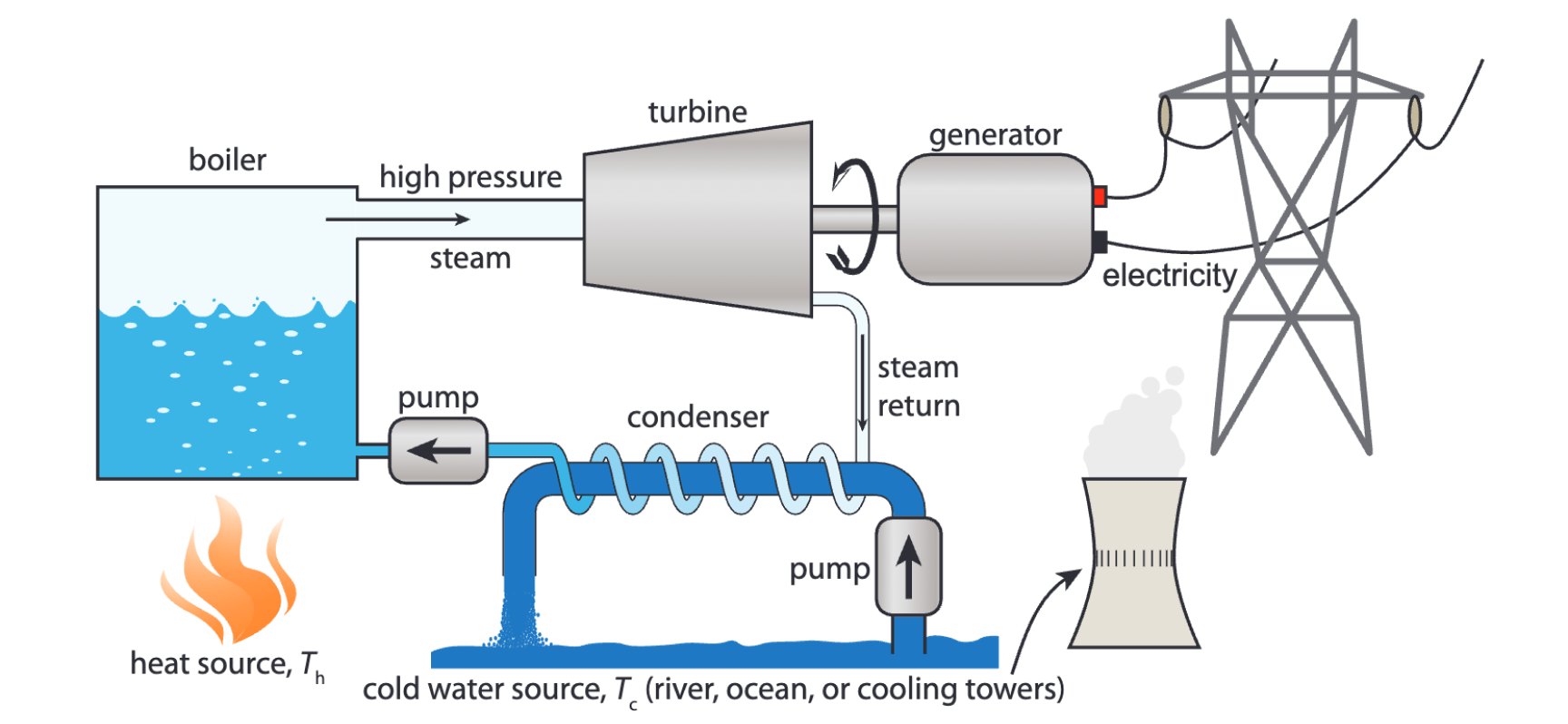
typical structure of a heat based power plant[2]
Coal is created when organic matter from dead plants and animals collects deep underground in high pressure environments and gets compressed over time.
The organic matter that has created coal started to exist around the Paleozoic Era (also called the Carboniferous Era) which started ~360 million years ago During this time, large quantities of plants and animals collected near river basins, where where they died and got buried.
These collections of organic matter eventually formed into underground beds called peat beds. As the peat got subjected to more pressure, it increasingly carbonized, creating more densely packed forms of coal over time.
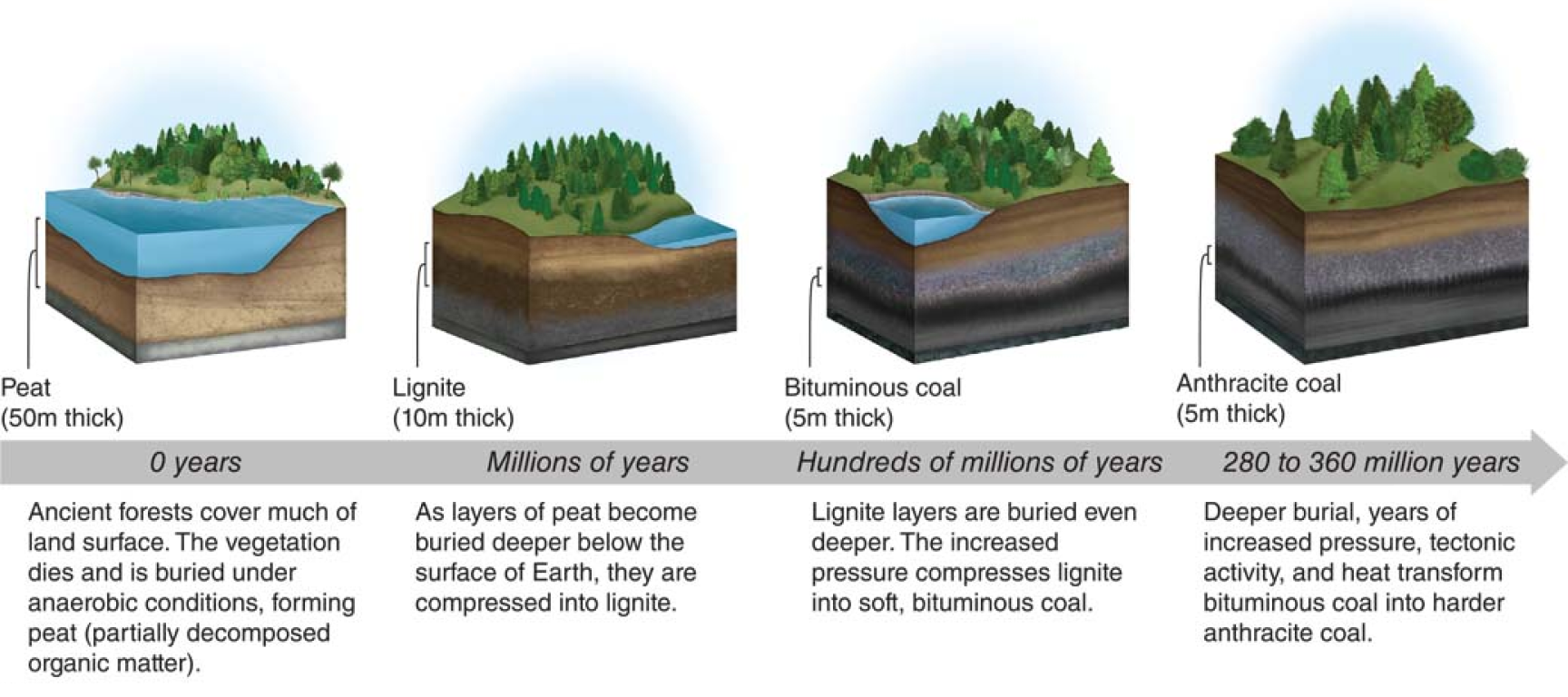
coal formation over time[3]
Aside: Coal grades
Lignite and sub-bituminous coal are the first types of coal that form, after ~100-250 million years.
They have a relatively low energy density, have high moisture, and are cheap, so they're cost effective for electricity generation.
Anthracite and bituminous coal are the highest quality coal forms with the highest carbon content and energy density. They take far more pressure and time to form - current deposits are ~300 million years old.
These coal grades are much more expensive so they're primarily used for industrial heating where their high energy density is valuable.
Bituminous coal is particularly critical in the production of steel, where it's turned into coking coal with low moisture and used in industrial processes.
Coal is generally purchased by the ton. Different grades of coal differ significantly in price and energy density. On average, one ton of coal contains ~2,200 kWh, enough to power ~73 homes for a day.
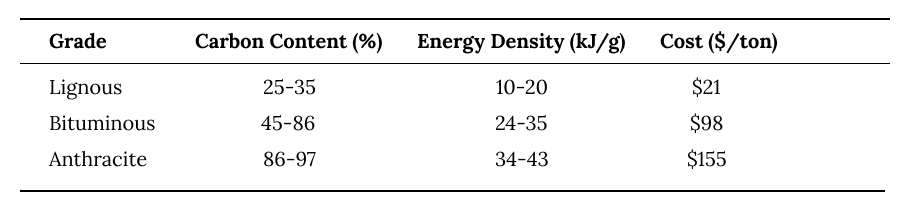
coal grades by quality and price
Coal beds exist on every continent and are relatively abundant compared with other fossil fuels, so most large countries are able to mine their own coal reserves rather than trading coal internationally.
They're also easy to find compared with oil and natural gas deposits. Exploratory drilling and seismic studies are used to evaluate new locations for coal mines.
Currently, there are an estimated 1.1 trillion tons of proven coal reserves in the world which is enough to last another 132 years at our current global consumption level.
Oil
Petroleum, also known as crude oil, is the source of almost all fuel products in the world like gasoline, diesel, and kerosene, as well as many other materials like tar, asphalt, and plastics.
Modern transportation is defined by its use of oil.
In fact, the mainstream adoption of the automobile with Henry Ford's model T is partly due to his use of an oil based engine. This cheaper form of energy offered a more economical fuel compared with the expensive electric automobiles that dominated the market at his time (the original cars were actually EVs).
In 2022, oil made up ~40% of global energy consumption, most of which came from transportation
Petroleum consists of hydrocarbon chains of varying lengths, which can be combusted with oxygen, in order to produce carbon-dioxide, water, and heat. This reaction produces more heat than coal, so oil is more energy dense.
oil combustion reaction
Crude oil forms in a process similar to coal.
Organic matter has to get buried 2-4 km underground in oxygen deprived environments. Then the pressure cracks long hydrocarbon chains in this matter into varying lengths, producing petroleum inside porous rock.
To keep this oil from escaping, an impermeable cap-rock needs to be present on top of the organic matter deposit.
All these constraints combine to make the formation of oil deposits rare. In fact, if you choose a random location on the planet to drill, there would be an 0.01% chance of finding an oil deposit.
Because oil is harder to extract and more energy dense than coal, it's also significantly more expensive. A barrel of oil costs around $70 on average today, and contains 1,700 kWh, enough to power ~56 homes for a day.
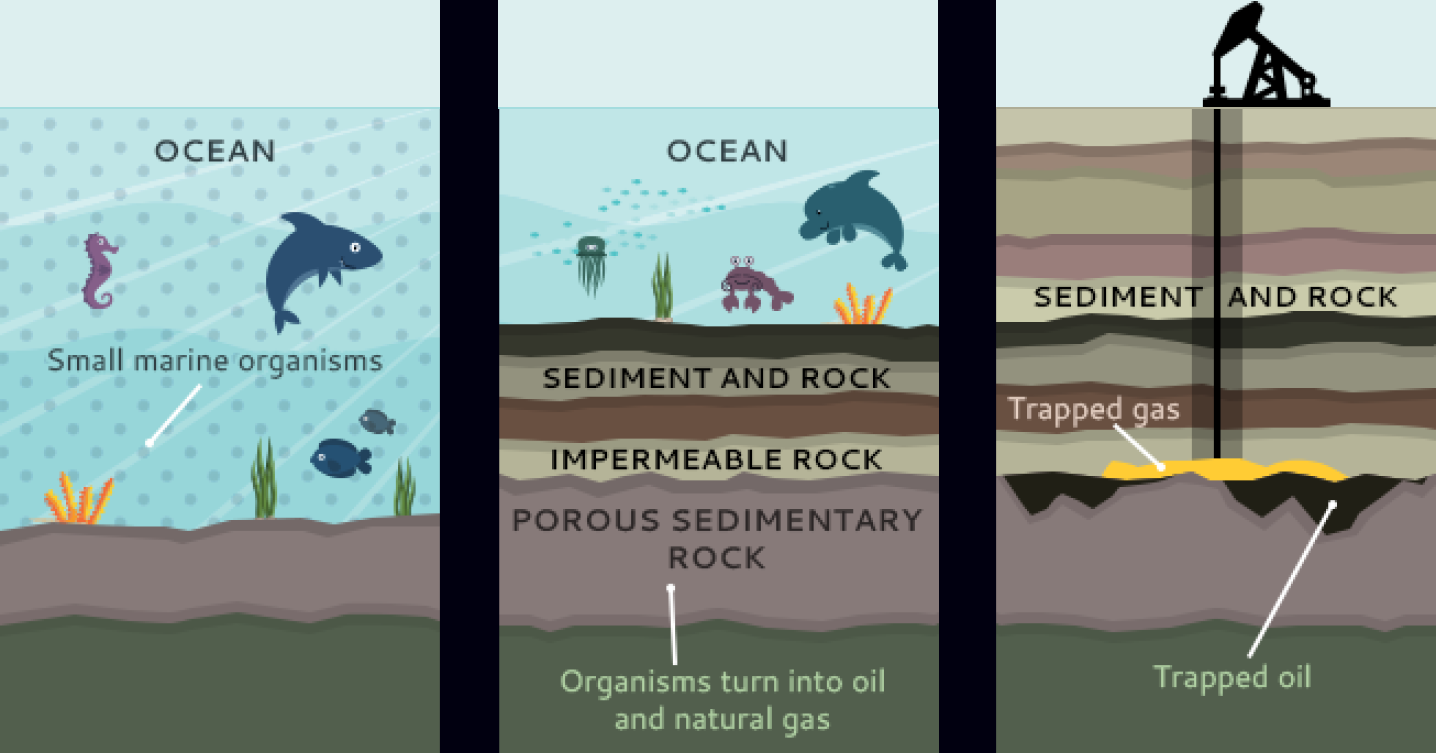
oil formation over time
Aside: Oil extraction and fracking
Early oil wells, discovered in the late 1800s, were easy to extract from due to their high pressures. When we drilled into them, pressure pushed oil to naturally emerge to the surface.
Over time, oil extraction has gotten much harder. We've depleted most of the high pressure oil reserves, so modern oil reserves are lower pressure.
Because of this, we have to extract the oil out of its rock either by using pumps or injecting liquid.
One of the most popular methods of oil extraction is hydraulic fracturing, known as fracking. This strategy shoots highly pressurized fluid into existing cracks below the earth's surface near oil reserves. The fluid expands the cracks, causing oil to flow from the surrounding rock.
This method has gotten especially popular in the US, which got two thirds of its oil from fracking in 2022. Fracking can cause significant downstream damage due to drinking water contamination and oil spills, making it controversial.
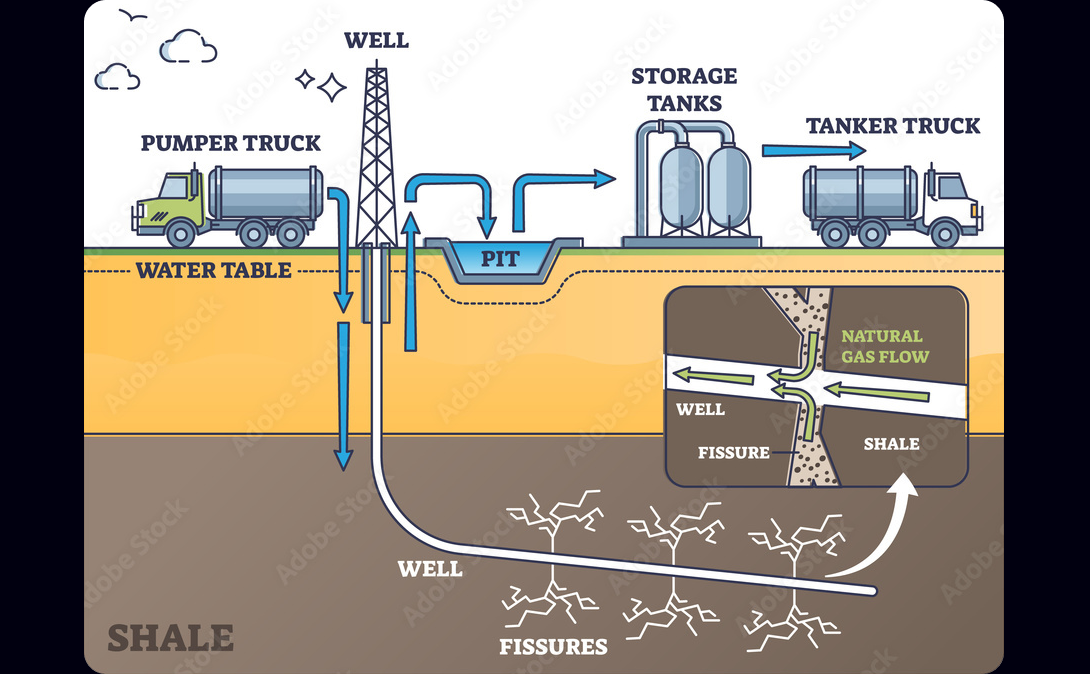
hydraulic fracturing to extract oil
Aside: Oil refining
After extraction, petroleum then has to go to a refining facility where the different hydrocarbon chain lengths within it are separated out.
Petroleum with higher sulfur content can damage the refining equipment and reduce yield of products after refining.
Low sulfur petroleum is considered "sweet" and offers much more efficient conversion.
The refining process heats the petroleum, and the differing boiling points of various hydrocarbon chains cause the separate products to rise to different heights in the reactor, allowing them to be separated.
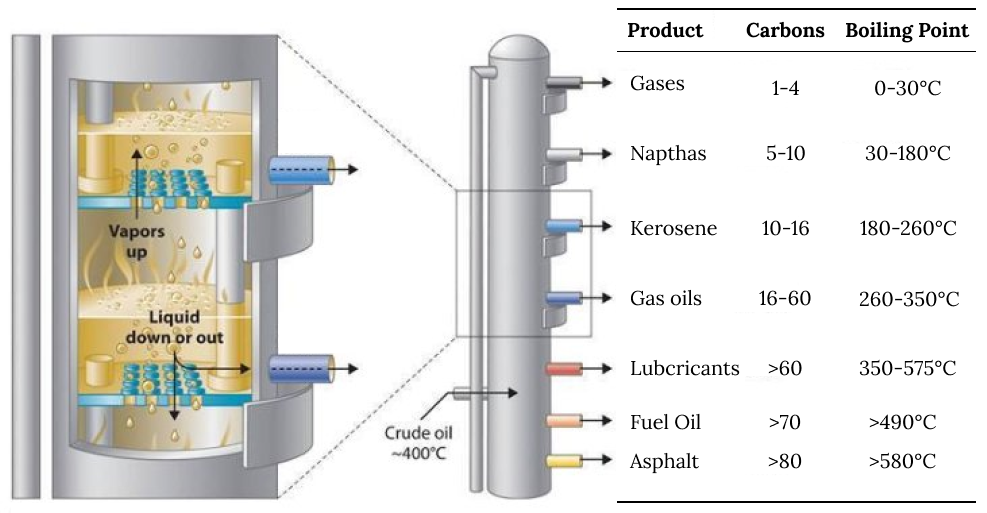
oil refining using a distillation tower
Once these chains are separated, they can be sold as different products. 92% of these products end up getting used as fuel, while 8% go toward chemical products that go into materials like tar, plastics, and lubricants.
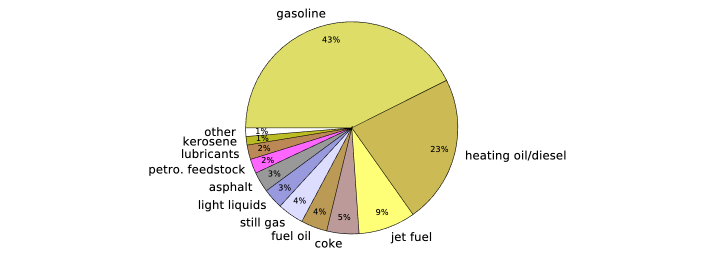
share of oil products after refining
The different petroleum products used for fuel all defer in their energy densities based on the lengths of the hydrocarbon chains they're made of.
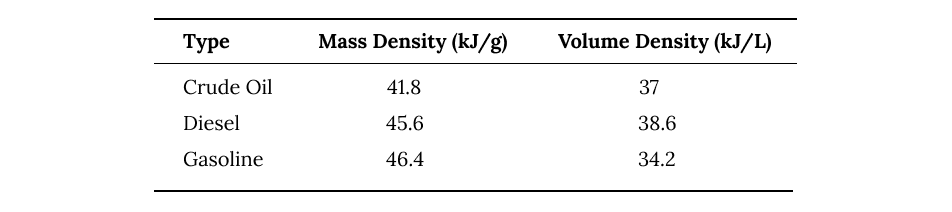
energy densities of different petroleum based fuels
Given the rarity of oils, and the fact that it takes >10 million years for oil to form, our present rate of burning through global oil reserves is unsustainable.
We have an estimated 1.6 billion barrels of oil in proven reserves. This would last us ~47 years at current consumption levels. The energy cost of extracting a barrel of oil has also increased by >10x since the 1800s.
Natural Gas
Natural gas is also found with many crude oil deposits.
It's created by the exact same sources of organic material, and is the result of hydrocarbon chains cracking repeatedly until they reach their smallest possible form: methane ().
Natural gas is much harder to handle since it's in gaseous form and must be highly pressurized during transportation. In Part IV, we'll see the distribution methods we've developed to mitigate this challenge.
In 2022, natural gas made up ~16.7% of global energy usage, and ~23% of electricity generation
Natural gas uses a combustion reaction like coal and oil, producing the most energy out of the three.
natural gas combustion reaction
Natural gas is a far more flexible electricity production source than coal and oil, owed to the invention of the combined-cycle turbine that is now used in many natural gas power plants.
This system uses heated and compressed natural gas to spin one gas-powered turbine, and then uses the exhaust from this turbine to heat water into steam, spinning another steam-powered turbine.
This process of recapturing the heat from natural gas to create steam is much more energy efficient and fast to startup compared with coal.
In Part IV, we'll see that this flexibility is particularly useful for balancing load on the electrical grid.
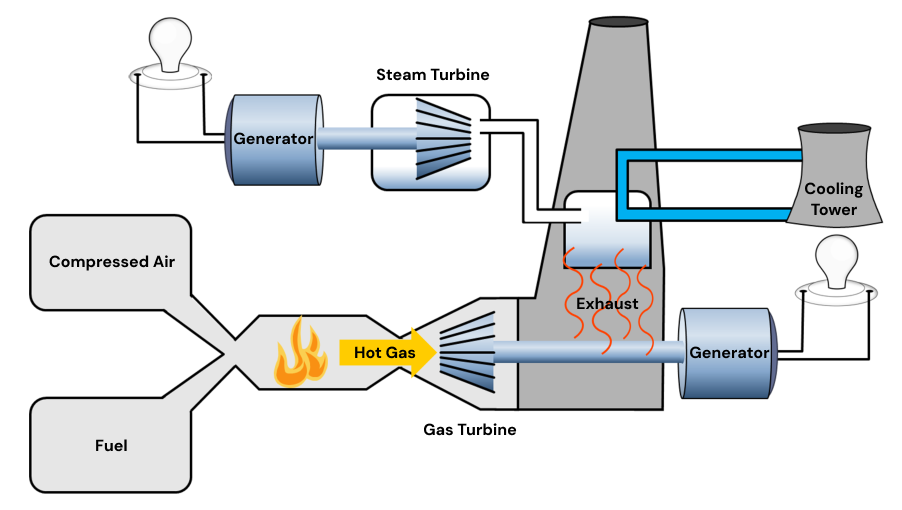
basic structure of a combined-cycle natural gas turbine
Natural gas is also commonly used for residential heating due to the efficiency of natural gas furnaces and the convenience of natural gas piping to homes, compared with the need for the transportation of coal and oil.
Natural gas is purchased in measurements of metric million British thermal units (mmBtu), which is roughly equivalent to 293 kWh, enough to power 9 homes for a day. 1 mmBtu of natural gas currently costs ~$2-3.
We have ~52 years of proven natural gas reserves remaining given present consumption levels.
Outlook on Fossil Fuels
Fossil fuels have been our primary energy source for the past 3 centuries and enabled the economic growth that has created modern society.
Their value can be attributed to their high energy densities, relative safety to burn compared with other fuels (fossil fuels catch on fire, while more reactive fuels can explode), and their relative abundance.
In the near-term, our primary concern with fossil fuels is their high emissions of green-house gases when combusted.
By burning fossil fuels at scale, we change the composition of our atmosphere such that it traps more heat from the sun onto earth, which has many downstream consequences.
I won't focus on emissions much in this deep dive, since it has already been heavily covered in other places, and a thorough breakdown of emissions would double the length of each section.
In the long-term, there's an even more fundamental problem with fossil fuels: our global fossil fuel reserves are finite.
Current estimates tell us that our fossil fuel reserves will be fully deployed in ~50-150 years given current energy demands.
These are likely underestimates since we often find new reserves.
However, this is balanced out by the fact that our energy demands are increasing at an accelerating rate, so assuming constant energy demand in these calculations misses the full picture.
Whether fossil fuel reserves are depleted in 100 or 500 years may not matter to the current generations, but the fact is that humanity will soon have to figure out how to create larger amounts of energy from sources that will last us much farther into the future.
For these reasons, the world has collectively agreed that it wants to move away from its dependence on fossil fuels toward renewables. As we'll see in Part VII, this transition has already started and is progressing faster than expected.
Renewables
Biofuel
The renewable energy source that most resembles fossil fuels is biological energy, which comes in solid form, known as biomass, and liquid form, known as biofuel.
In 2022, ~9.3% of global energy consumption and ~2.2% of global electricity generation came from some form of biomass or biofuel.
Biomass and biofuel have energy stored in the form of sugar and fat that can then be combusted with oxygen to produce heat, just like fossil fuels.
combustion reaction of ethanol, a type of biofuel
The energy in biofuel all originates from plants' conversion of solar energy into chemical energy through photosynthesis.
Plants use the radiant energy from photons to combine carbon and water, producing sugar to store energy and oxygen as a biproduct.
chemical reaction of photosynthesis
Photosynthesis captures between ~0.01-6% of the energy in sunlight, depending on the plant.
Algae is on the most efficient end of this, which is why algal biofuel has been experimented with. However, most efforts to develop this type of fuel, including those of ExxonMobil, have now been stopped due to impracticality.
Wood and animal dung are the most common forms of biomass used for combustion. They're most used in developing nations where access to fossil fuels is expensive and limited.
Ethanol is the most common form of biofuel, made from the fermentation of plant matter using bacteria and yeast. Most ethanol today is made from corn starch.
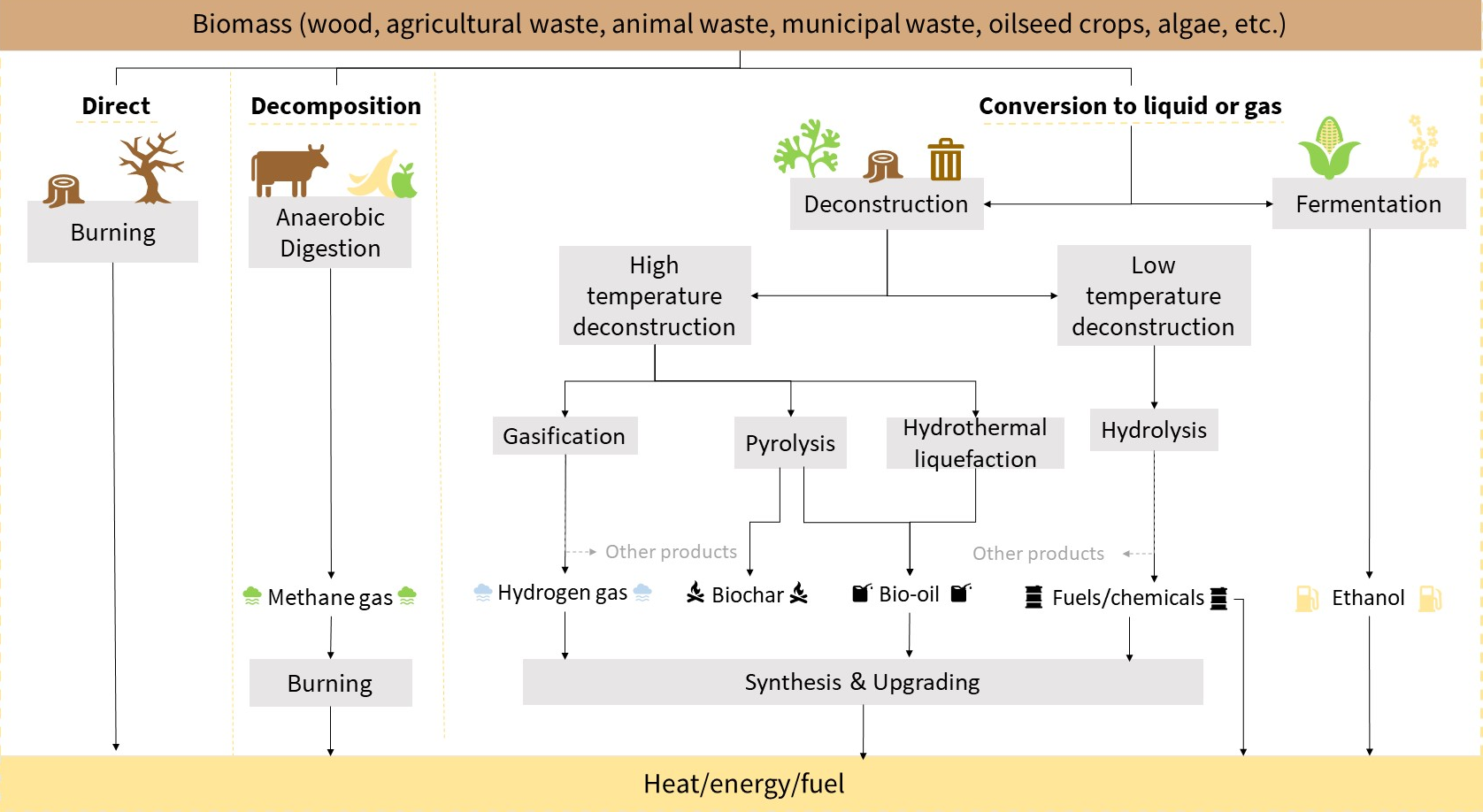
different biomass to biofuel conversion processes[5]
Ethanol often gets combined with gasoline in various mixtures to produce slightly more renewable, though less efficient, fuels for transportation. The most popular mixture is Gasohol E10 which consists of 10% ethanol and 90% gasoline. Biofuels can also be combined with petroleum products to create biodiesel.
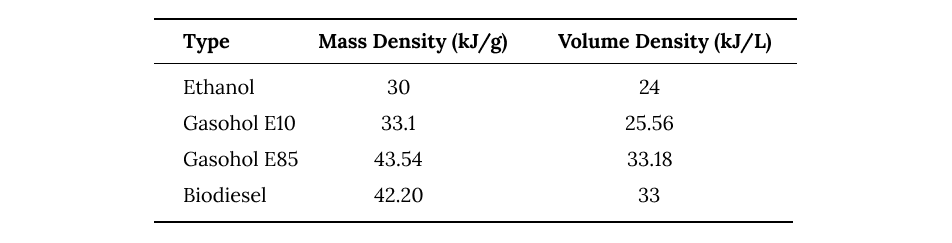
energy density of different biofuels
Could ethanol replace oil consumption?
Given an average total solar input of 250 W per square meter on the planet, and assuming a 1.5% energy conversion efficiency of photosynthesis in corn over a 5 month growing season, each square meter of corn would generate .
We would then need a 2,220 km by 2,200 km plot of land growing corn to produce enough ethanol to replace global oil consumption.
This would be an area roughly half the size of the US, which is clearly infeasible.
Plant-based biofuel is technically renewable since plants grow back.
There's currently an estimated 100 TW of biomass on the planet, meaning that plants on earth are collectively capturing 100 TJ of energy from the sun every second. In fact, this is the total net energy input to all biological systems on the planet.
We would then have to burn 18% of this biomass every year to capture the 18 TW needed to meet global energy demands. Burning such a large portion of earth's biomass annually is impractical.
Biofuel is highly energy inefficient due to the high energy demands of our agricultural system.
For example, for every 1 J we spend producing ethanol, we get 1.2 J back, which is unlikely to scale to the needs of the entire transportation industry.
Overall, we can see that biofuels are not energy efficient or abundant enough to meet our current global energy demands.
Hydroelectric
Capturing the kinetic energy of flowing water is one of the first methods of energy conversion that humans have used, with early water mills developed in ancient Mesopotamia and China in the 4th century BCE.
Modern hydroelectric systems use large dams to keep water raised at a height above sea level.
This water then contains gravitational potential energy which increases pressure at the bottom of the dam, allowing water to flow through and spin turbines that generate electricity.
In 2022, ~16% of global electricity generation came from hydroelectric.
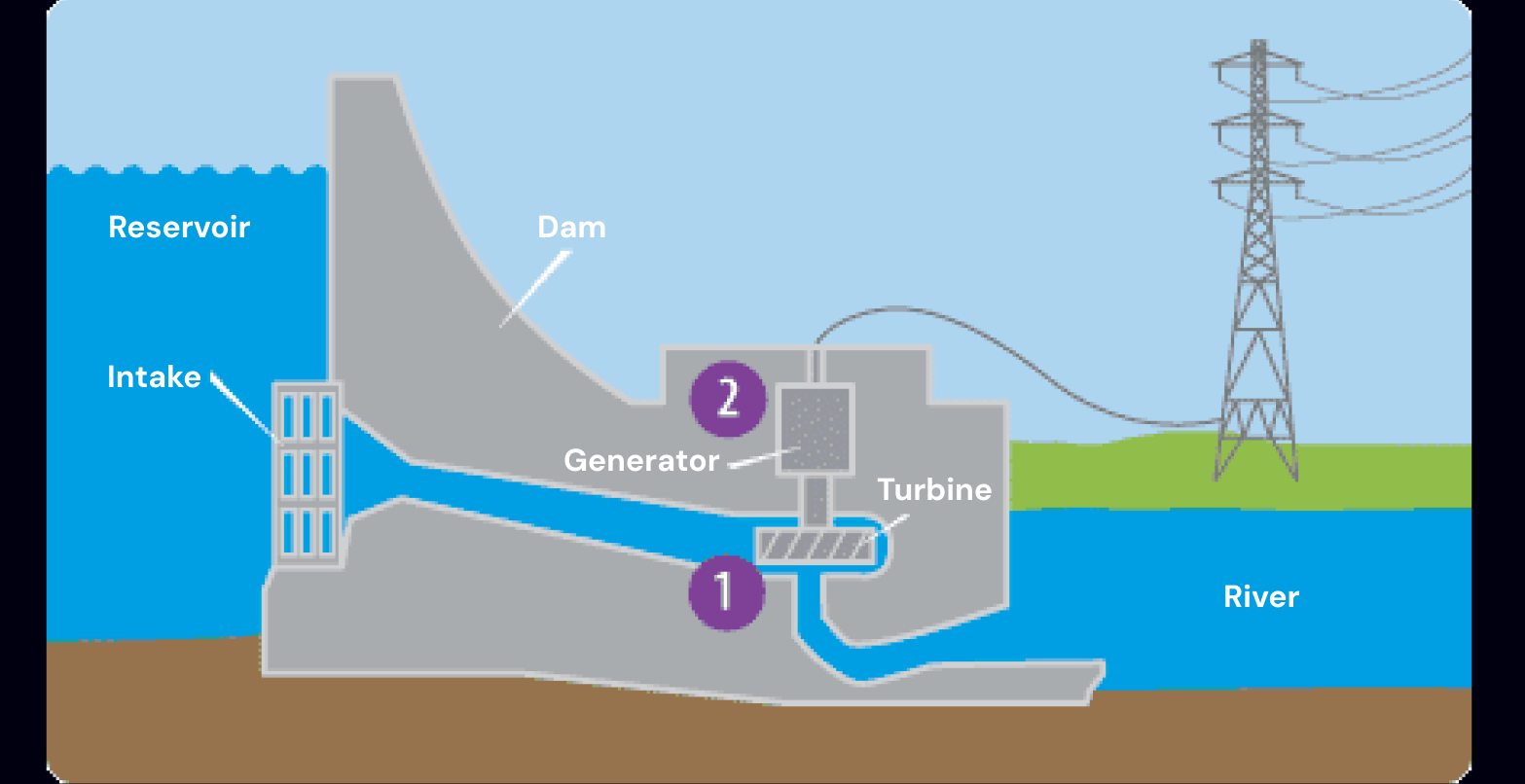
basic structure of a hydroelectric power plant
It seems like the energy in this process originates from gravitational potential energy naturally stored in water.
But how does this water get gravitational potential energy in the first place?
If we've set up a dam with a finite supply of water behind it, this water should eventually get depleted over time as it flows through the dam.
In reality, energy from the sun is constantly heating water from the ocean, causing it to evaporate and consense into clouds.
Eventually, this water rains down onto land, and runs off into streams and then larger rivers that can be dammed to produce electricity.
So the net energy input that powers hydroelectric is actually coming from the sun.
Aside: How energy efficient is hydroelectric?
If we look at the full cycle of this energy conversion process, we see that most of the energy input by the sun is lost in elevating the water into clouds, the air resistance as rain falls, and the energy lost by the water that never ends up in rivers with dams.
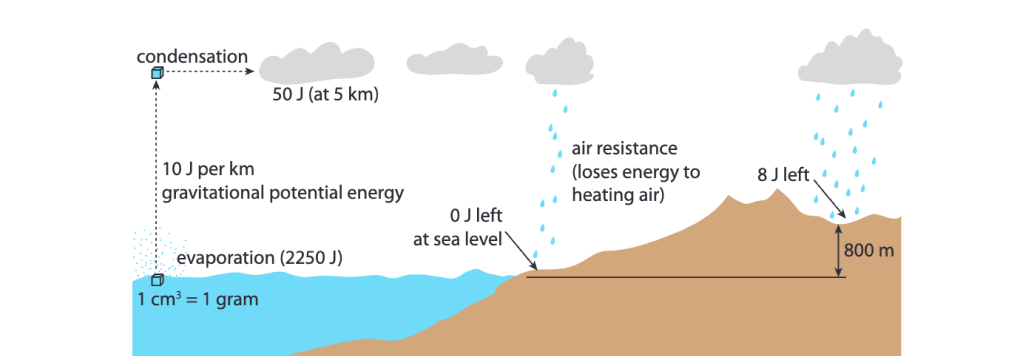
the energy conversion of hydroelectric[6]
For every 2000 J initially input by the sun to evaporate water, we get less than 2 J back from hydroelectric. So this is energy conversion process is <0.1% energy efficient.
Based on the total amount of dammable water in the world, there's an estimated ~2.5 TW of total viable hydroelectric power globally.
So hydroelectric will clearly never be able to scale to support our global energy needs.
Wind
Wind is a similarly old technology, first harnessed by sailboats around 3500 BCE and by the wind powered vessels that enabled the beginning of global trade around 300 BCE.
In 2022, ~7.5% of global electricity generation was from the wind, with this number quickly rising due to a global adoption and build-out of new wind generation capacity.
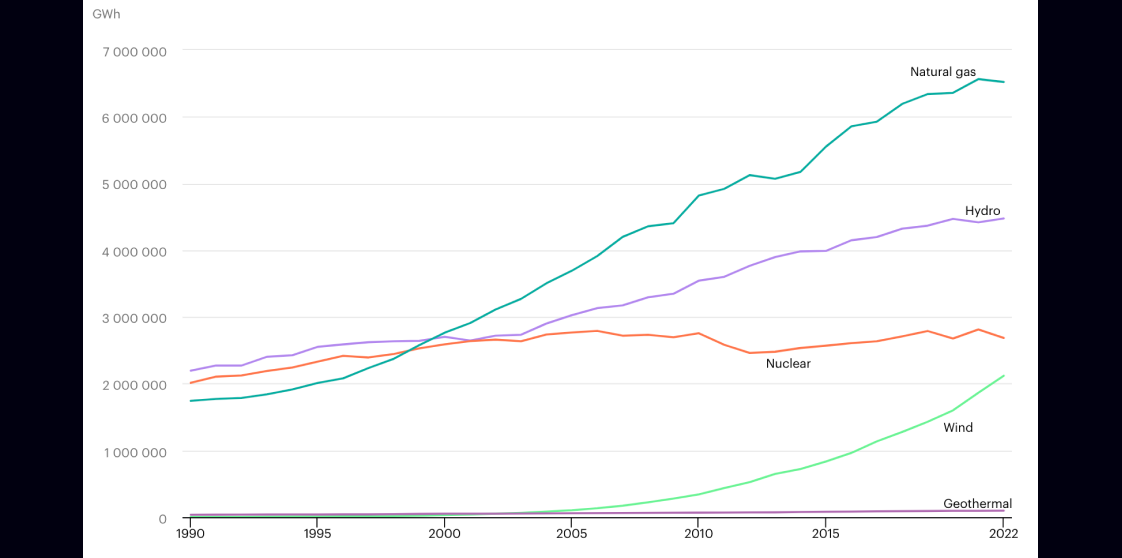
wind generation is growing quickly compared with other sources[7]
Wind turbines capture the kinetic energy of wind particles and convert it into rotational kinetic energy.
This works due to the curvature of the rotor blades, causing passing wind to apply lift and drag forces to the turbine and make it spin. In this sense, the rotor blades work like the wing of an airplane.
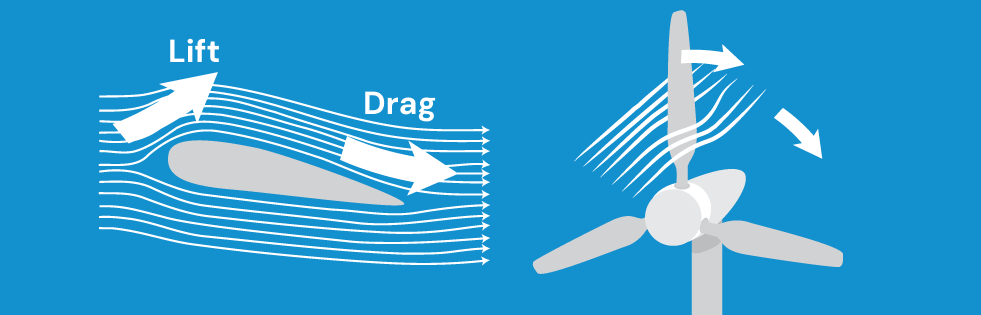
the forces acting on a wind turbine
The net energy input for wind also originates from the sun.
When the sun heats up different sections of air unevenly, they create differences in pressure, causing air to flow from high to low pressure areas. This air flow is what we call wind.
Aside: Why offshore wind?
We can understand why offshore wind has gotten popular by analyzing the actual power output of a wind turbine.
Let's look at the cylinder of air that transfers some of it's kinetic energy to the turbine.
The cross-section area of the cylinder is equal to with representing the length of a single turbine blade.
The length of the cylinder in a unit of time with a wind speed is .
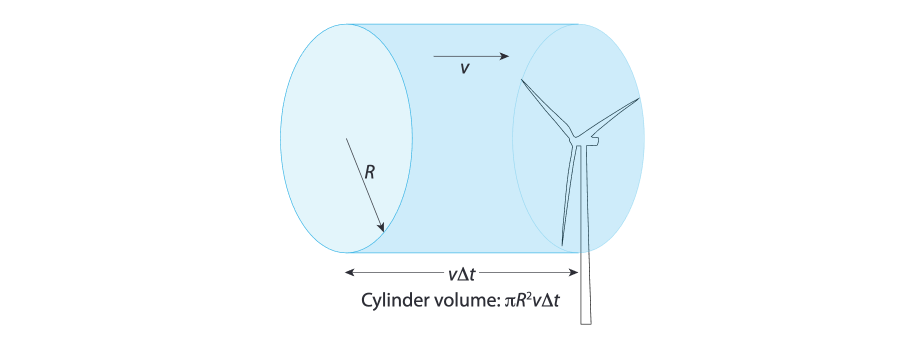
the column of air that interacts with a wind turbine[8]
So the total volume of the cylinder is , and with an air mass of we get the total mass of the cylinder .
Then we can calculate the total kinetic energy captured by the wind turbine with .
Dividing both sides by , we get energy over time on the left which gives us power, and we see that the total power produced by the turbine is .
So we see that the total power output depends on the square of the turbine length, and the cube of the wind speed.
Higher wind speeds will yield disproportionately higher power outputs.
This is part of why the idea of offshore wind is so popular: higher wind speeds off the coast lead to much higher power outputs.
However, the cost of actually building offshore wind infrastructure currently means that the net cost of electricity produced by offshore wind is often higher than onshore wind farms.
Despite this, governments are pursuing offshore wind due to the convenience of building larger turbines off the shore and away from residential areas with higher wind speeds that generate more power.
Wind turbines can't convert 100% of the kinetic energy of the surrounding air to power. Capturing 100% of energy from the wind would mean stopping the air in front of the turbine, which would divert other air to flow around the turbine, preventing further power generation.
So a balance must be maintained to allow wind to continuously flow. In practice, wind turbines can't pass ~40-50% conversion efficiency from kinetic energy to power output.
Additionally, the intermittency of wind means that wind turbines aren't always operating at their maximum capacity.
Deployed turbines operate at ~33% capacity factor, meaning that they produce 33% of the equivalent power output that they could if they were constantly operating at their limit.
It's estimated that the total kinetic energy of our entire atmosphere is ~350-700 TW.
There are a variety of estimates on how much of this energy can actually be captured, based on turbine efficiencies and practical limitations on where wind power plants can be built. Most estimates are in the 10-20 TW range and some even go as low as 2-3 TW.
Our current global wind production is already ~1 TW and growing quickly, which makes me skeptical of the lower estimates.
Regardless, we can see that while wind may be able to support current global energy needs if all practical wind capacity were deployed, there isn't much room for it to grow to meet our exponentially increasing future energy demands.
Fission
Nuclear energy is the most complex energy source that we have learned to control.
The first fission reactor was invented in 1942. By 2022, nuclear made up ~7.5% of global electricity generation and 4% of total energy production.
New nuclear generation stagnated until very recently due to public perception and regulatory issues. In Part VII, we'll cover recent developments in fission reactor design that have started to build more interest in nuclear deployment again.
Nuclear power plants use fission reactions that split heavy elements into lighter ones, producing heat. They use this heat to power a steam turbine and generate electricity, just like any other traditional power plant.
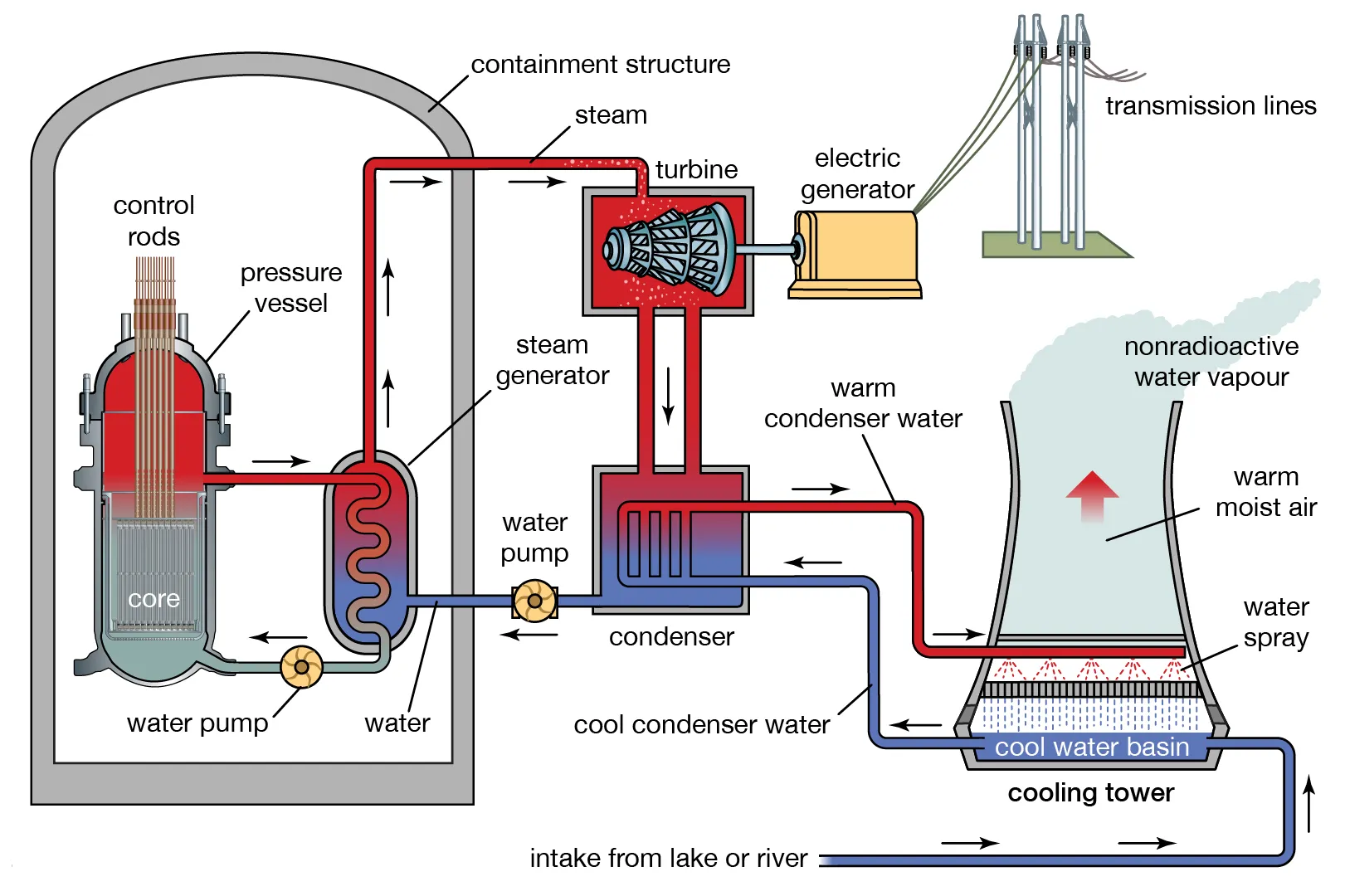
basic structure of a fission power plant[9]
Fission produces heat by releasing energy stored in atomic nuclei.
In order to understand this, we have to take a closer look at the properties of these nuclei.
Atomic nuclei consist of protons, which are positively charged, and neutrons, which have no charge.
In order to bind these particles together, something needs to overcome the fact that the positively charged protons all repel each other due to electromagnetism.
The strong nuclear force is the force that opposes this effect, binding protons and neutrons so strongly when they are close enough to each other that they overcome the repulsion of electromagnetism.
Aside: Radioactive decay
This tension between the forces in atomic nuclei is the source of radioactivity.
Only certain quantities of protons and neutrons are able to stay stably in the same configuration.
We can see the combinations of protons and neutrons that yield stable atoms below.
Notice that as elements get heavier with more protons, they need a higher ratio of neutrons to protons in order to counteract the repulsive forces between protons.
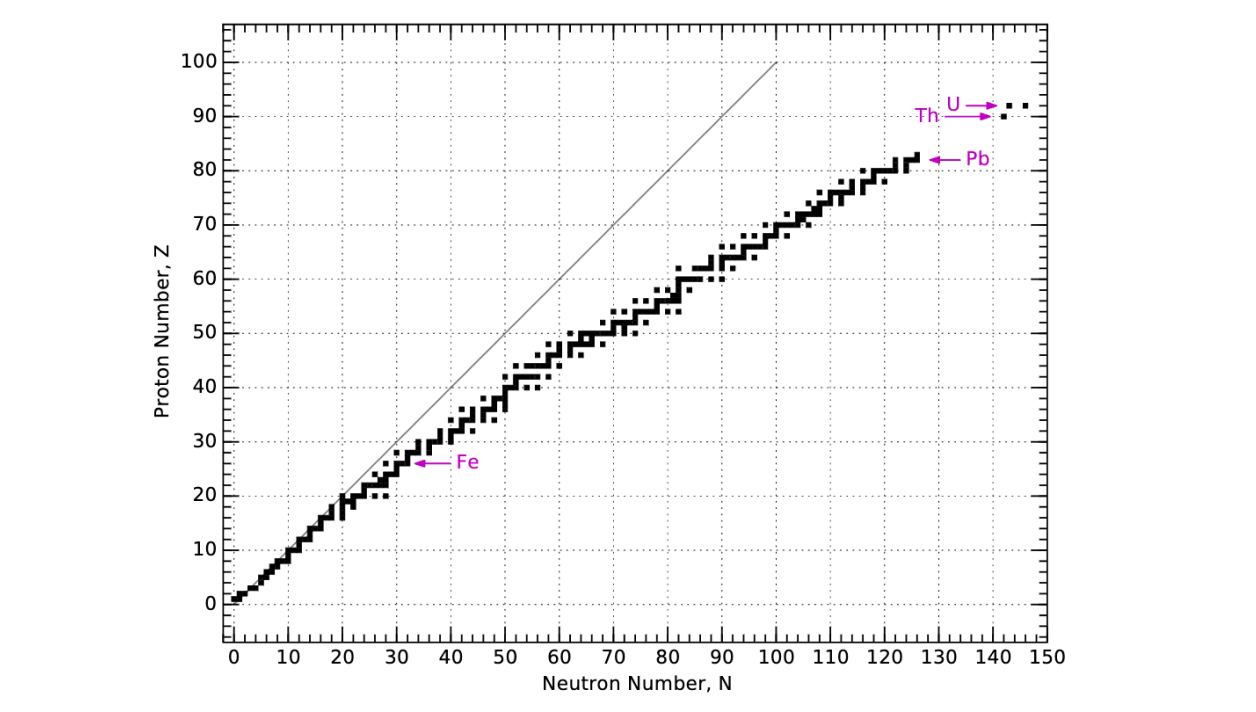
stable atoms by proton and neutron compositions[10]
Configurations of protons and neutrons that are unstable, known as isotopes, have to change their forms to become stable.
They accomplish this through radioactive decay, where they transform or emit particles to alter their compositions and become stable.
There are only a few ways that particles can decay:
- Alpha decay: () Atoms release two protons and two neutrons together in the form of a Helium nucleus.
- Beta-minus decay: () A neutron in the nucleus converts into a proton, and the atom emits an electron.
- Beta-plus decay: () A proton in the nucleus converts into a neutron, and the atom emits a positron (which is the opposite of an electron).
- Gamma decay: () The nucleus emits a high-energy photon called a gamma ray.
Gamma rays are particularly dangerous for biological life forms because they can rip through DNA at high speeds, causing mutations.
We can see how certain isotopes decay using these methods in the following chart.
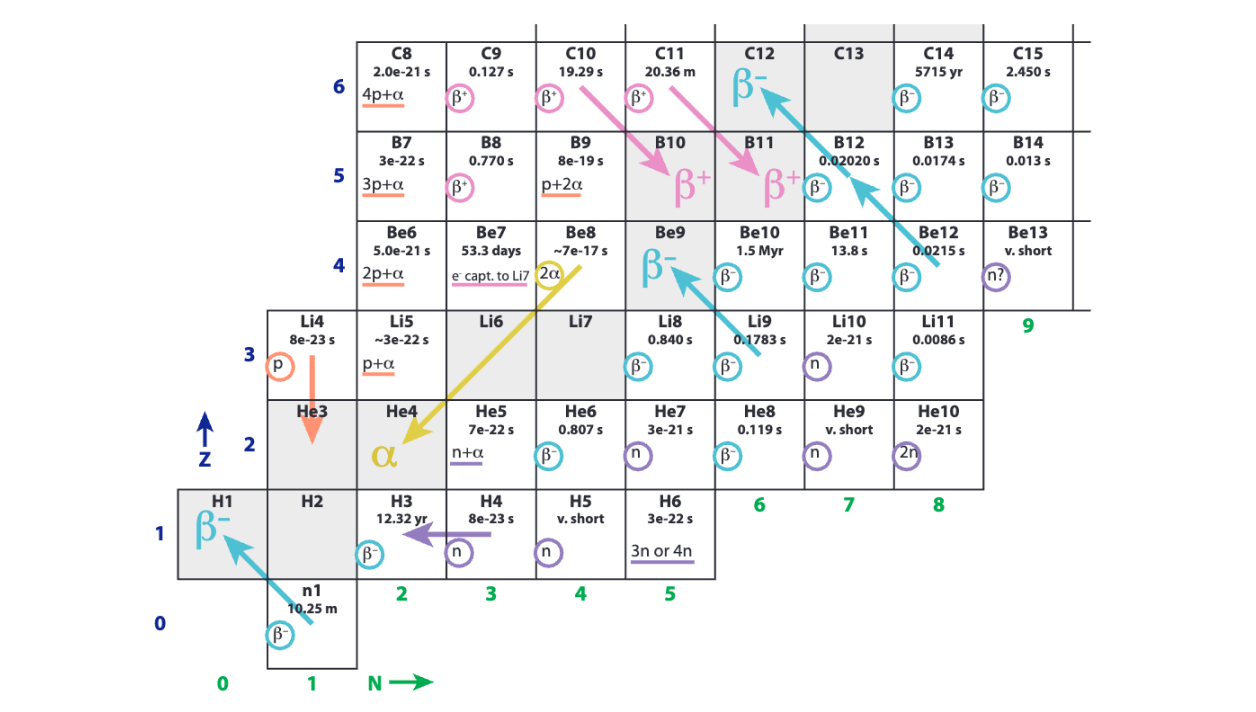
fission decay patterns for different isotopes[11]
In fission reactions, stable heavy atoms often split into lighter nuclei that are unstable isotopes. Then, the isotopes follow these radioactive decay patterns to get to stable elements.
Some of these isotopes emit gamma rays, which is why fission waste is dangerous and must be carefully disposed of.
Now we can take a look at the energy that's present in atomic nuclei.
Einstein's famous equation, tells us that all energy actually has a small amount of mass.
This means that whenever any energy has entered a system, the total mass in the system has also increased.
The mass is usually just too small for us to ever notice. For example, 1 J of energy has a mass of
However, at the scale of an atom, the changes in mass due to energy are actually noticeable.
For example, if we consider an individual nucleus, it will take a massive amount of energy to split this nucleus into its individual protons and neutrons.
If we supply this energy and split the atom into individual particles, we will notice that the sum of the weight of all the particles is more than the original weight of the atomic nucleus.
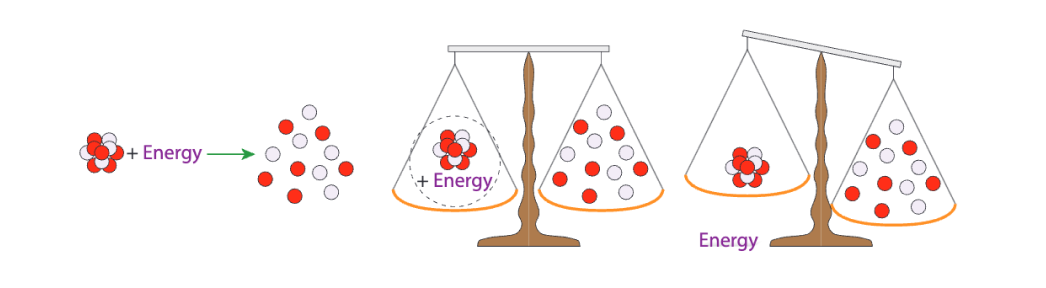
visualizing atomic binding energy[12]
How is this possible? It turns out that because of how much energy we had to put in, the energy has now perceptibly changed the weight of the particles.
Now if we could put this atom back together, it's mass would decrease again as it would release that same amount of energy in the process.
We can call this energy the binding energy of the atom. You can think of it as the amount of energy that would get released if you were to form an atomic nucleus from just its protons and neutrons.
Let's take a look at the binding energies of different elements. This chart shows us that hydrogen (H) on the left has the lowest binding energy of any element. Then the highest binding energy element is iron (Fe). Then binding energies start to decrease again toward heavier elements.
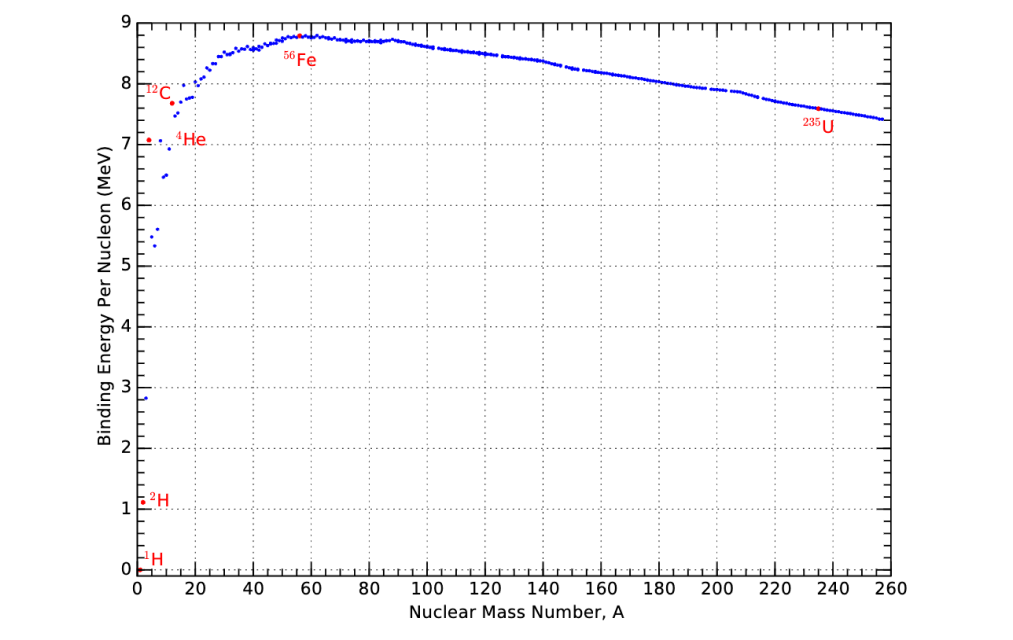
the binding energies of different atoms[13]
I find that looking at this graph upside down is the most intuitive way to visualize what's happening in nuclear reactions. We can think of the bottom of the pit as the atom that has released all the energy it possibly can, whereas other elements can move downhill in order to release more energy.
Then we see that heavy elements on the right can move downhill and release energy by splitting into lighter elements.
We also see that combining lighter elements like hydrogen offers the steepest drop, representing the most energy released from the reaction.
This is why fusion, which combines lighter elements together, provides so much more energy than fission, which splits heavy elements.
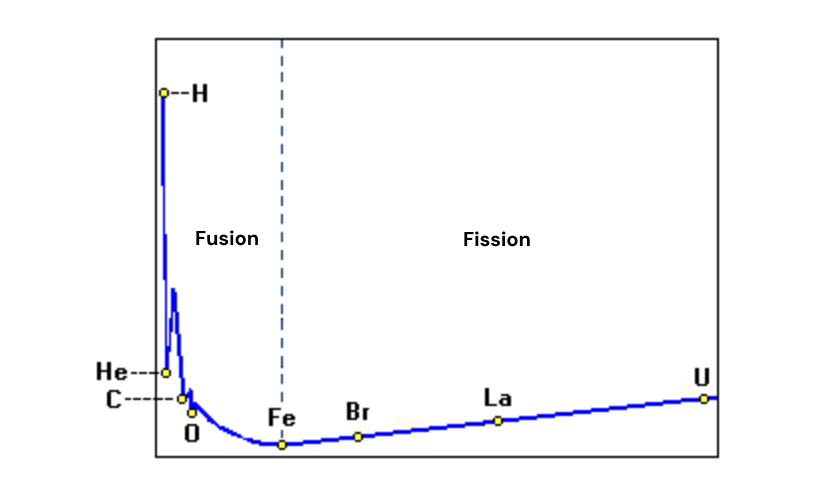
visualizing the binding energy chart upside down
Now that we understand where the energy in fusion comes from, we can see that fission is the only energy production method we have today that doesn't depend on energy from the sun. Instead, it uses energy deposited in the atomic nuclei of heavy elements by old fusion reactions.
In practice, the energy present in heavy elements usually originated from astrophysical anomalies like supernovae and merging neutron stars.
Let's take a look at the actual fission reactions that have enabled us to harness nuclear energy.
There are only 3 elements we've identified that can be practically used in fission reactors: Uranium-233, Uranium-235, and Plutonium-239. Out of these elements, only Uranium-235 is actually found in nature.
So let's understand how the Uranium-235 fission reaction works.
Uranium-235 is perfect for fission because it's very easy to split.
If we get a slow moving neutron to stick onto the surface of the U-235 nucleus, it will cause the nucleus to instantly become unstable and split into two smaller elements, releasing 2 neutrons and 2 gamma rays in the process.
These neutrons can then be used to create more fission reactions in nearby U-235 nuclei, creating a chain reaction that releases energy.
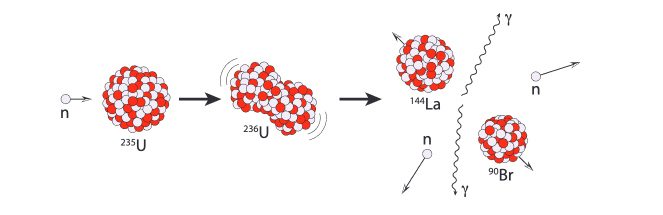
fission reaction with Uranium-235[14]
The neutrons that are shot out from split U-235 nuclei are moving too fast to stick to other U-235 nuclei.
To solve this, we use moderators like Boron that absorb the speed of neutrons. This slows the neutrons down enough to bump into new atoms and cause more fission reactions.
Nuclear reactors use rods of U-235 fuel with Boron moderator rods, known as control rods, in a reaction chamber.
The control rods are the primary means of controlling the rate of fission reactions. If reactions are happening too quickly, the control rods are inserted further into the fissile material to slow them down.
Nuclear meltdowns like Three Mile Island, Chernobyl, and Fukushima occur when these reactions run out of control. We'll discuss nuclear reactor safety systems more in Part VII.
Heat from the reactor chamber is then used to convert water into steam, which is used to generate electricity.
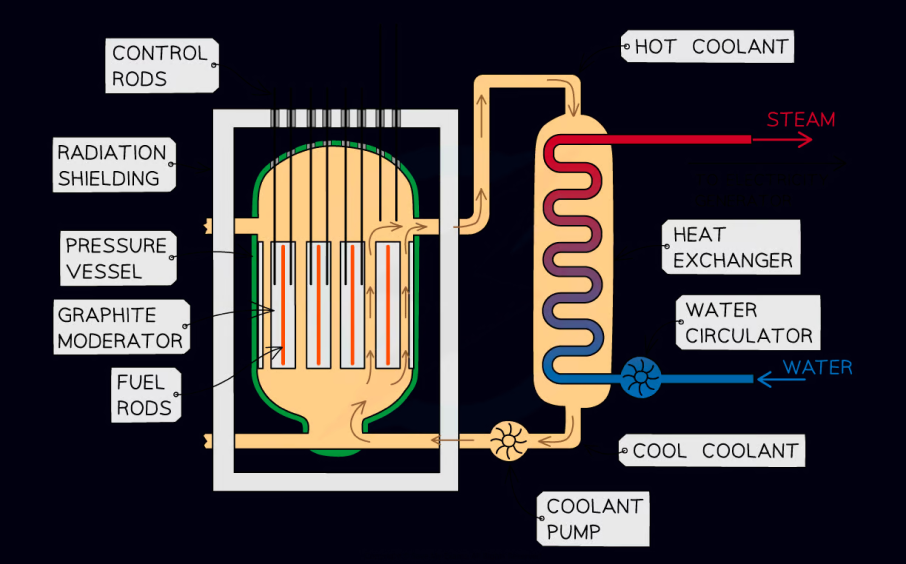
basic structure of a fission reactor chamber
Aside: Spent fuel waste disposal
When fission occurs, U-235 usually splits into unstable isotopes that undergo radioactive decay to turn into stable elements.
This decay can happen over the course of thousands of years, meaning nuclear waste stays radioactive and dangerous.
We need safe disposal methods to ensure that this radioactive waste can decay safely over time without damaging the biosphere or harming people.
There are 3 different waste-disposal methods for nuclear waste.
First, nuclear fuel that has just been taken out of the reactor is kept in spent fuel pools for ~5 years. The water in these pools cools down the hot fuel and prevents radiation from escaping.
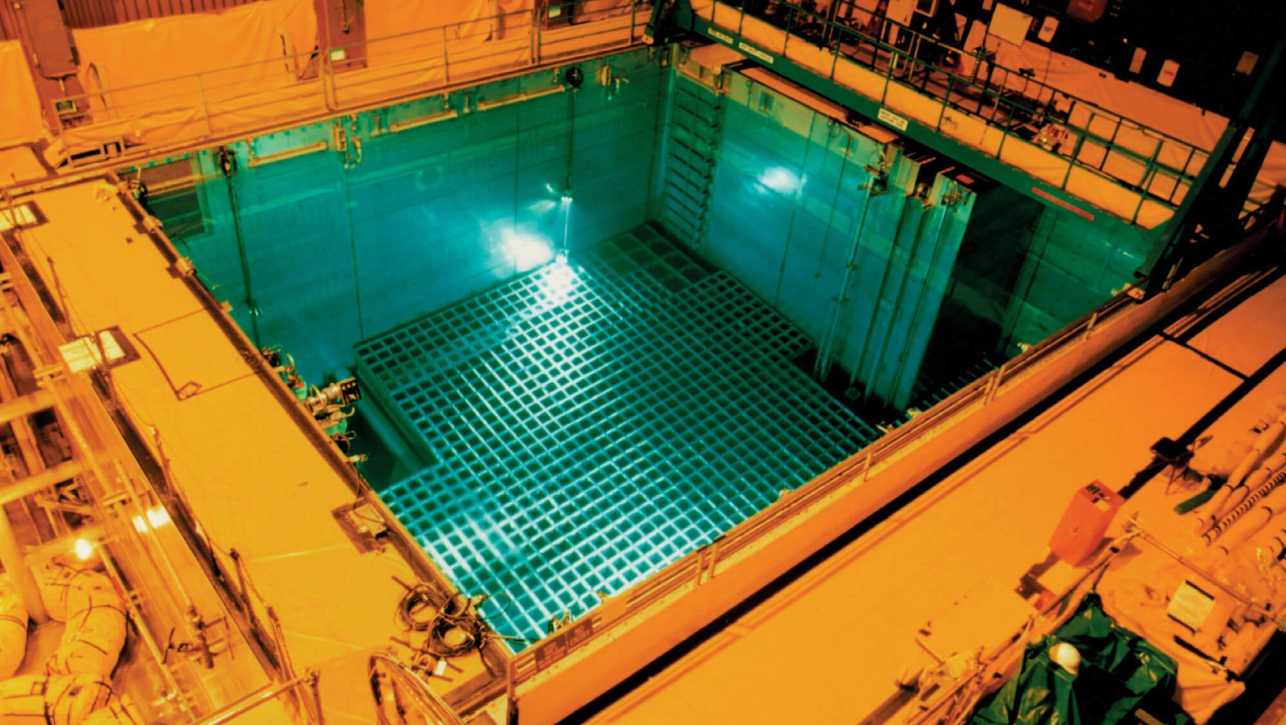
a spent fuel pool for nuclear waste disposal
Then, fuel is transferred into dry cask storage where it's wrapped in thick casks of steel and concrete, which provide physical shielding from radiation. These casks are then stored on-site in nuclear reactor facilities.
Dry casks can safely store nuclear waste for decades, so they work for now but are only a temporary solution.
The long-term disposal for nuclear waste involves burying dry casks 500-1000 m underground in deep geological repositories (DGRs). These are underground formations in clay and granite that are stable and prevent radiation from escaping.
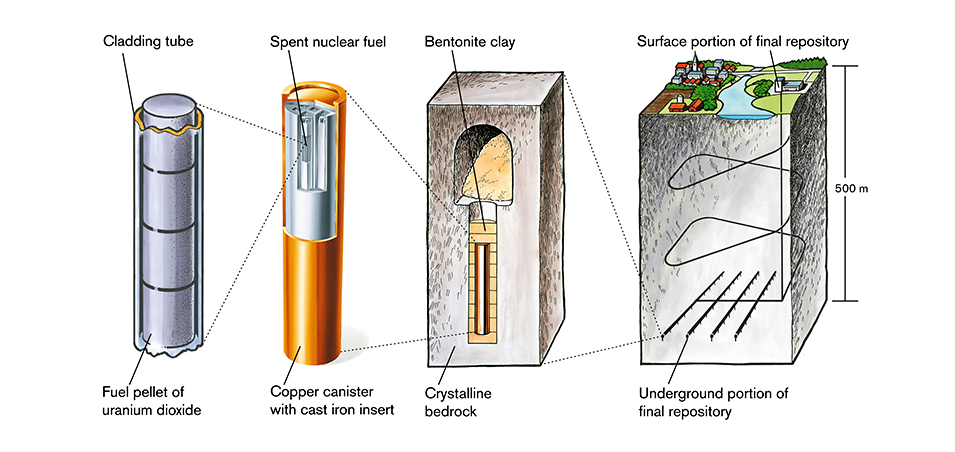
the ideal long-term disposal process with dry casks and DGRs[15]
There are no existing DGRs for burying nuclear waste due to the difficulty of surveying and constructing safe facilities deep underground.
This means that all nuclear waste today is in temporary storage.
We saw that fuels like coal, oil, and natural gas usually have energy densities ~30-60 kJ/g.
Meanwhile, nuclear fuel like U-235 has ~70-80 million kJ/g. This difference makes it clear how much more energy dense nuclear fuel is compared to any other fuel source.
Naturally occuring U-238 is not a particularly abundant metal, but the fissile U-235 is even rarer.
Uranium is never produced in the fusion reactions of stars, so could only have been produced by rare astrophysical cataclysms as we mentioned earlier. This is why it's so rare.
We currently have ~7.6 million metric tonnes of proven uranium reserves.
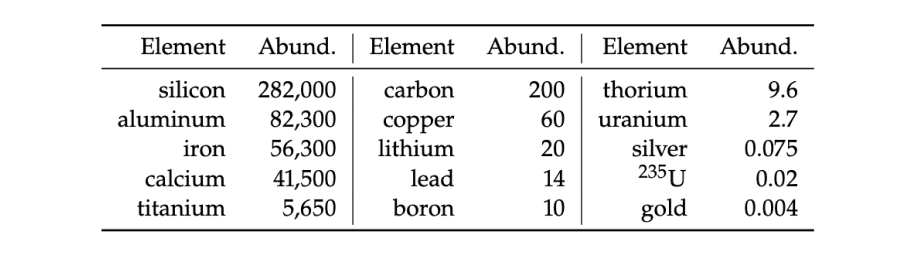
natural abundance of different metals in parts per million
Out of these reserves, 99.3% of this is U-238 which is not fissile, with only 0.7% being U-235. We then have to extract the U-235 from U-238 using uranium enrichment, which isn't a 100% efficient extraction process.
Factoring in these inefficiencies, our current uranium reserves would provide ~90 years more of fission energy at current usage rates, or 4 more years of energy if we produce all 18 TW of global energy demand with U-235 fission.
To address this scarcity of fissile fuel, we've come up with ways to artificially create fissile Uranium-233 and Plutonium-239 from more abundant elements like thorium by intentionally inducing these elements to undergo radioactive decay. This process is known as breeding
Reactors that use these fuels are called breeder reactors. The successful deployment of these methods would increase the abundance of useable nuclear fuel to last >20,000 years.
In Part VII, we'll see that the first ever breeder reactor was successfully deployed in 2023.
Overall, we see that nuclear fission fuel is by far the most dense energy source we've learned to control, and that if we can scale breeder reactors, fission will offer us abundant energy far into the future.
Fusion
As we saw above, fusion uses the same source of energy as fission: the binding energy present in atomic nuclei.
Instead of splitting heavy elements like U-235, fusion opts to combine the lightest elements like different hydrogen isotopes in order to release much more energy.
The hydrogen that fusion depends on is far more abundant than fission fuels, which is a big reason why fusion is so appealing.
There are currently two main chemical reactions for fusion that are being pursued, the fusion of two deuterium atoms (the hydrogen isotope with a neutron) known as D-D fusion, or the fusion of one deuterium and one tritium (the hydrogen isotope with 2 neutrons) atom known as D-T fusion.
fusion chemical reactions
In comparison to the 70-80 million kJ/g we saw with fission, D-T fusion releases ~337 million kJ/g, and D-D fusion releases ~639 million kJ/g.
However, in order to successfully achieve fusion, we have to stick protons together.
This requires the protons to travel at ~7% the speed of light.
Most particles can only really reach these speeds at ~1 billion degrees with the conditions we have on earth, with hydrogen isotopes able to reach fusion at lower temperatures.
Specifically, D-D fusion starts to occur around 100 million degrees, and D-T fusion around 45 million degrees. We have chosen to primarily develop D-T fusion reactors first since reaching 45 million degrees is easier.
Meanwhile, the sun is able to achieve fusion at "only" ~16 million degrees because it's so massive that gravitational forces contribute to the fusion environment.
Deuterium is present as 0.0115% of naturally occurring hydrogen.
With the current amount of hydrogen present in the ocean, we have enough fuel for 60 billion years of D-D fusion.
Meanwhile, tritium is not naturally occurring. We create it by adding a neutron to a lithium atom and waiting for it to decay.
Assuming all current lithium reserves went toward making tritium (which is certainly not possible since lithium is in high demand for batteries), we would have ~6,500 years of fuel for D-T fusion.
Aside: Fusion heating methods
There are 3 major heating methods used in fusion reactors to achieve fusion temperatures of >45 million degrees.
First, deuterium and tritium gas is initially ionized by sending electric current through it, turning it into a plasma (a gas that becomes electrically conductive due to freed electrons). This process is known as ohmic heating.
Once the plasma is present, high-frequency electromagnetic waves are sent out, resonating with ions and electrons in the plasma and further accelerating them. This is known as radiofrequency heating (RF).
In addition, high-energy particles are shot at the plasma, transferring their kinetic energy to the plasma particles through collisions. These injected particles are usually deuterium and tritium atoms that are neutrally charged, so they can pass through the magnetic field that contains the plasma. This is known as neutral beam-injection.
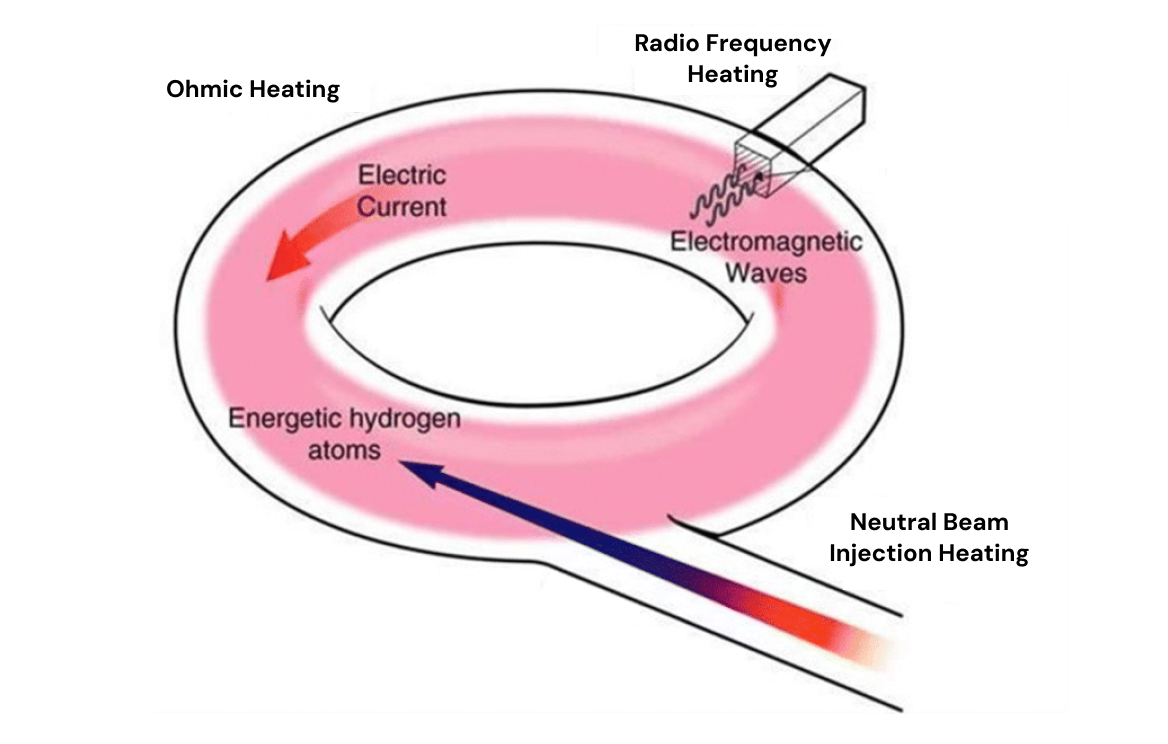
different forms of fusion heating
As soon as plasmas hit fusion temperatures with these 3 methods, D-T atoms within the plasma start to fuse, which further heats up the plasma in a process called self-heating.
Aside: Plasma containment methods
If heating is successful, then the main challenge becomes containing the plasma, which has now reached temperatures hotter than the sun.
The plasma has to completely avoid touching the walls of the containment vessel for fusion, or it will damage the walls and slow down the plasma, preventing it from reaching fusion temperatures.
There are currently two major containment methods being pursued.
The most popular approach uses magnetic confinement where extremely powerful magnets are used to bend the path of the plasmas in the shape of a circular toroid, which looks like a donut.
This works because the plasma is made up of fast moving charges, so they will feel strong effects from the magnets due to the Lorentz force, and will follow magnetic field lines.
Tokamaks, stellarators, and z-pinch fusors are all just different configurations of the fusion toroid and the magnetic fields going through it that control the flow of plasmas, so they never touch the edges of the device.
Many of the most popular fusion attempts, including the >$20 billion ITER fusion reactor and the most well funded fusion startup Commonwealth Fusion Systems (which has raised ~$2B so far) use tokamaks.
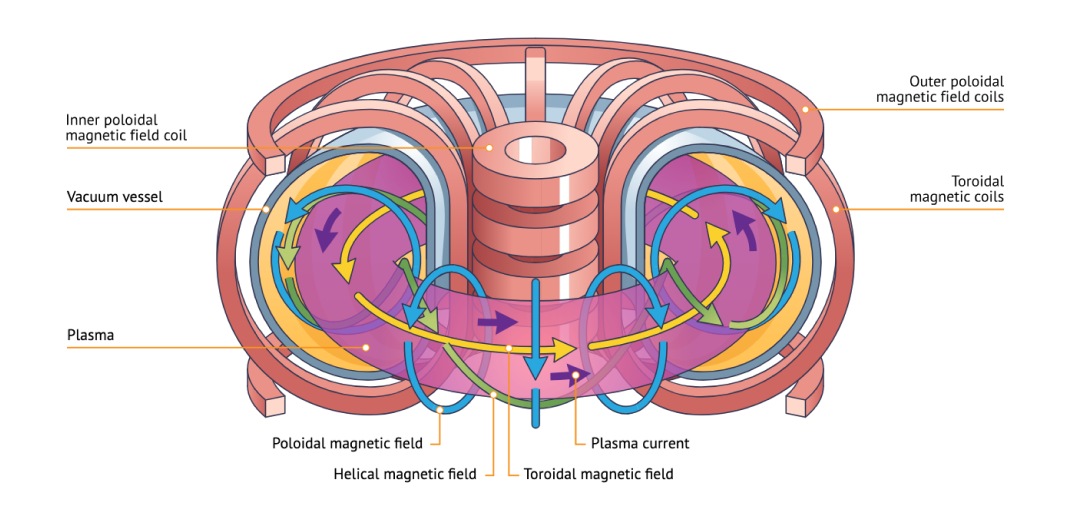
structure of a tokamak
Another magnetic confinement approach that has gotten popular recently is the use of the field-reverse configuration (FRC) where a magnetic field is induced in the shape of a toroid around a cylindrical plasma (instead of putting the plasma in a toroidal container), which also works to keep the plasma contained.
This approach is being used by TAE Technologies (which has raised ~$1B) and Helion Energy (which has raised ~600M).
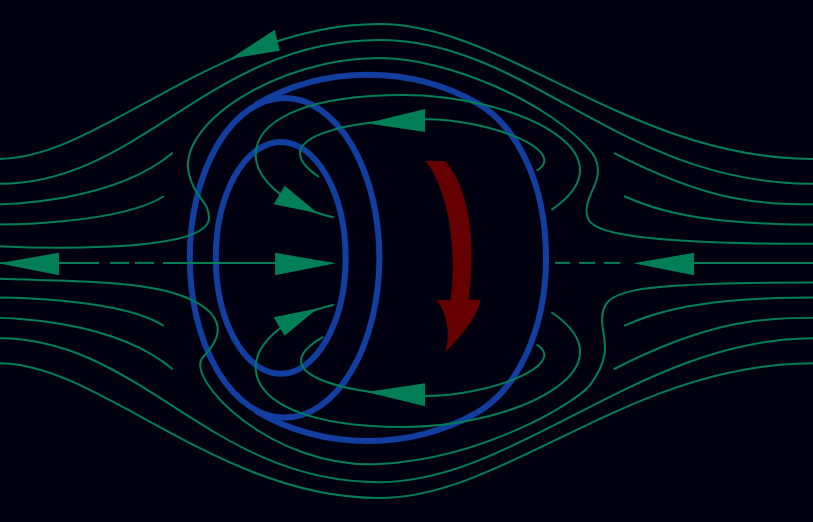
field reversed configuration (plasma in blue)
The other main type of plasma confinement is inertial confinement, where laser or ion beams are shot at the fuel, causing it to compress and heat up, making fusion far more likely.
Helion's approach uses something similar to this known as pulsed magnetic compression where they use magnetic fields to further compress plasmas together, leading to fusion that pushes back on this compression and generates an induced current that can be directly captured.
Because of this, Helion's fusion reactor bypasses the need for the typical steam turbine setup to generate electricity.
In 2022, the National Ignition Facility (NIF) achieved the first fusion reaction that delivered more energy produced than was introduced in the fuel, with 2.05 MJ of fuel sent in, and 3.15 MJ returned. While this is an impressive milestone, the total net energy production of the entire reaction was still massively negative.
Few people are familiar enough with fusion systems to accurately predict how far we are from net electricity producing fusion. It's unlikely that even the leaders of top fusion companies can predict this with accuracy. I certainly won't attempt to discern between the varied predictions out there.
If we look to the past, we'll see justification for the common meme that fusion has been "10 years away for the past 50 years".
This is not a reflection of our lack of progress; we have been making progress. Instead, it reflects our uncertainty about how much work is left.
The bottom line is that fusion is really hard, requiring temperatures of 45 million degrees and magnets strong enough to lift an 100000-ton aircraft carrier out of the water (the strength of the magnet in ITER).
If we can solve D-D fusion, it will provide us with near limitless energy abundance. But fusion may represent the hardest engineering challenge that humanity has ever faced.
Solar
When we understand how hard fusion is, it's hard not to appreciate the fact that we have a fusion reactor in the sky that's already working and constantly sending 173,000 TW to earth's upper atmosphere, which is 10,000x more than our current global energy needs.
We also saw that all the other energy forms we use aside from nuclear energy derive their energy from the Sun.
In this sense, capturing energy from sunlight is the most direct way to access the net energy input to our planet.
In 2022, solar accounted for ~4.6% of global electricity generation. In 2023, solar accounted for ~5.5%.
That makes it the fastest growing electricity generation method we have today. We'll see in Part VII that it has consistently been exceeding adoption predictions for the past decade.
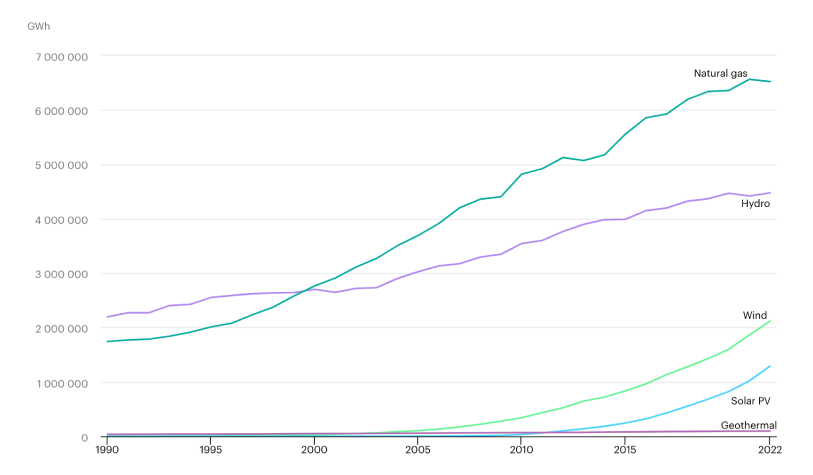
solar electricity generation growing quickly compared with other sources[7]
Let's look at how electricity production with solar panels works.
Aside: The energy emitted by the sun
First, we have to understand the energy contained in light emitted by the sun.
Light comes in the form of packets of energy called photons. Photons have an energy defined by Planck's constant , the speed of light and their wavelength defined by .
So shorter wavelengths of light like blue light carry more energy than longer wave lengths like red light. Specifically, a blue-green photon carries of energy.
(electron-volt) is the energy required to move an electron through an electric field of 1 volt, and is equivalent to (electron-volt).
Light is naturally emitted by bodies of mass as they get hot. This is known as black-body radiation.
The hotter they get, the more photons they emit (scaling with the fourth-power of temperature), and the shorter the wavelengths of light they emit on average.
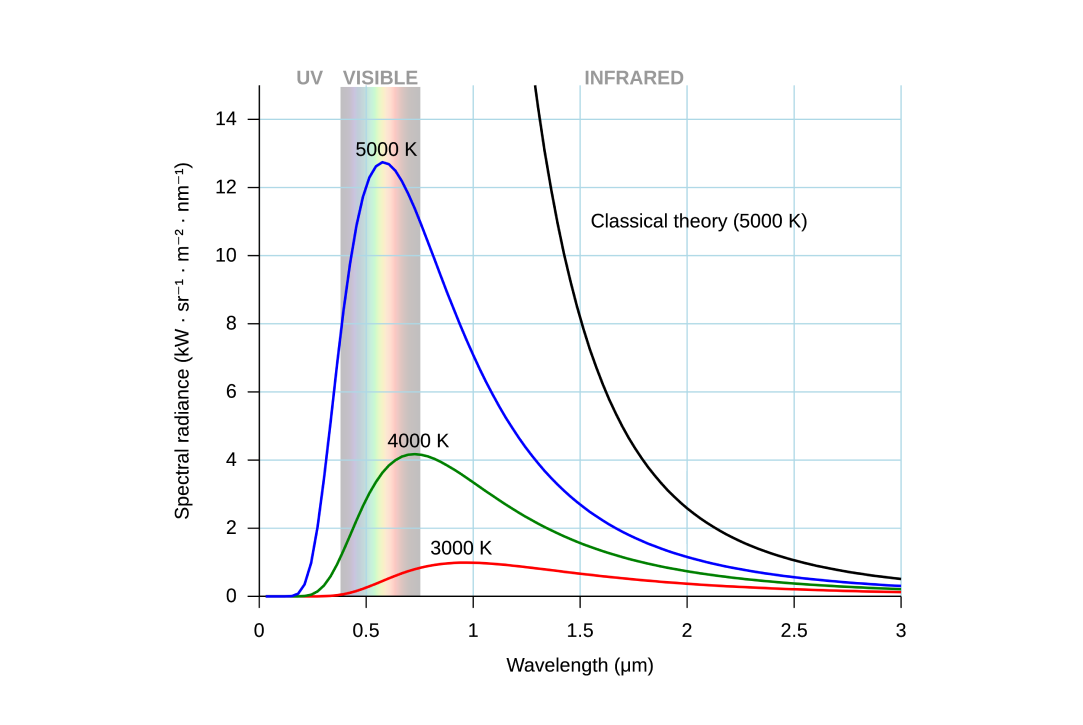
black-body radiation of masses of different temperatures[16]
Because the sun's surface is hot (~5800 K), it emits photons that are mainly visible light, with some UV and infrared light as well. All these emitted photons carry energy.
Photovoltaic cells (PVs) are able to capture part of the energy present in sunlight to generate a current.
Photovoltaics are composed of a layer of "p-doped" silicon with "holes" caused by a deficiency of electrons that allow free electrons to move around easily, and a layer of "n-doped" silicon with an excess of electrons.
These layers are separated by a junction where charges from each layer line up and create an electrical barrier that prevents electrons from moving from the p-layer to the n-layer. This is known as the p-n junction.
If an electron receives enough energy to move across the p-n junction from the p-layer into the n-layer, it will instantly be repelled by all the electrons in the n-layer toward metal contacts at the top, moving through an external circui to the p-layer and generating a current.
The goal of the photovoltaic cell is to get as many electrons as possible to complete this loop.
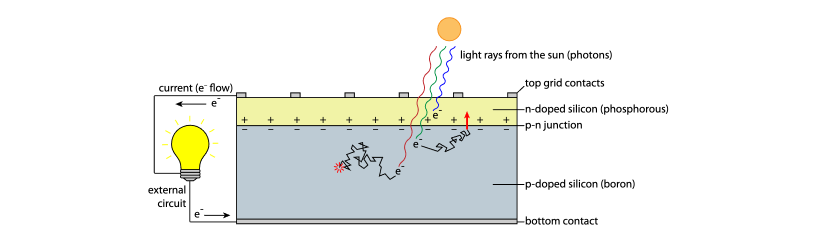
function of a solar photovoltaic cell[17]
Aside: How energy efficient are photovoltaic cells?
We can understand the energy efficiency of a PV by looking at what percent of the energy in incoming photons is captured by electrons.
Most of the electrons in the p-layer of a photovoltaic are stuck in the valence shells of the silicon atoms that make up the material.
Electrons in this layer need a 1.1 eV energy input to be freed from their valence shell. We say that these electrons have a band gap of 1.1 eV.
This means that any light with below 1.1 eV, including all infrared light, will pass through the PV without freeing any electrons. 23% of the photons that make it through the atmosphere pass through the PV like this.
On the other hand, photons with too much energy, like the blue-green photons with 2.5 eV, cause electrons to leave their valence shells with too much speed.
These electrons bounce around rapidly inside the PV dissipating energy as heat. 33% of the energy coming from photons is lost to heat because of this.
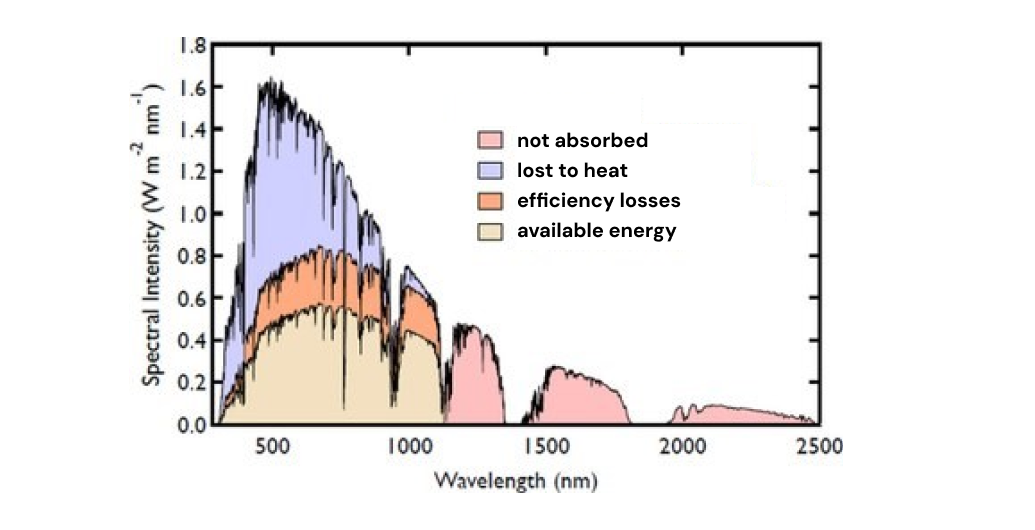
an example diagram of how different wavelengths of light interact with a PV cell
After a photon successfully jumps an electron out of its valence shell, the electrons randomly bounce around. Some of these electrons will accidentally fill holes caused by other freed electrons, and won't make it across the p-n junction.
Out of the total energy that reaches the solar panel in the form of photons, only 32% gets get trasferred to electrons that actually make it across the p-n junction and generate current.
Given imperfect energy conversion efficiencies, actual PV cells end up getting around 15-20% efficiency of converting the energy in photons to electricity.
The total power output of the sun is ~. By the time this sunlight gets to the earth, it will have spread out over a sphere with a radius of the distance from the earth to the sun, leaving us with around . This is known as the solar constant, which represents the total solar energy that arrives at our upper atmosphere.
Once this sunlight reaches the lower atmosphere, much of it will have been reflected by the air, leaving ~ varying with latitude and weather. This is known as the insolation of an area.
We can see the total insolation of different parts of the world and the US in the charts below. As expected, the total insolation near the equator is the highest, though the difference in the areas with the most and least insolation is only a factor of 2, meaning solar should be viable everywhere.
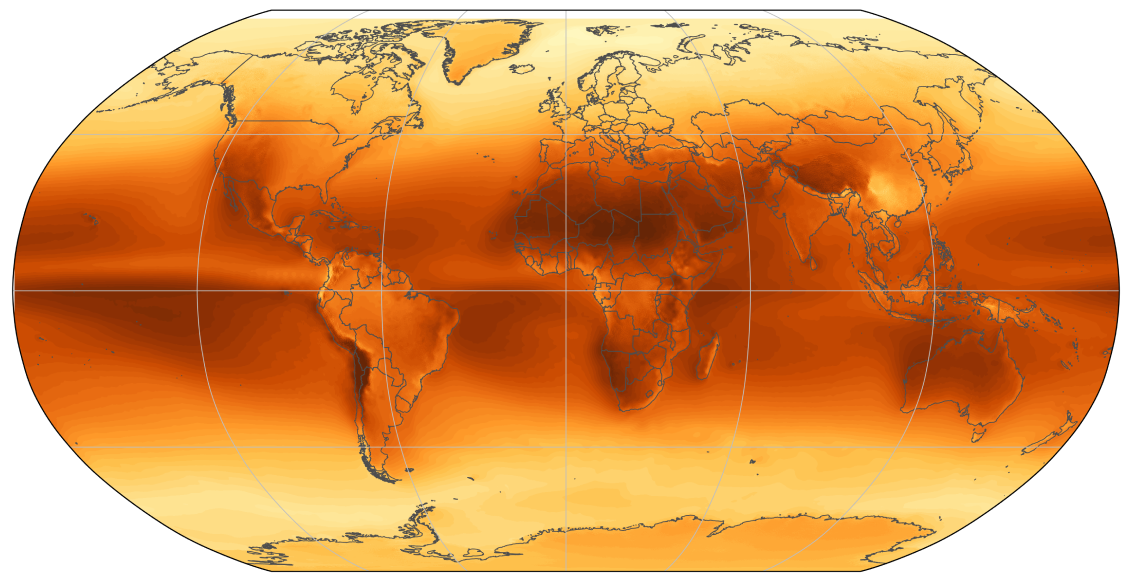
insolation map of the world[18]
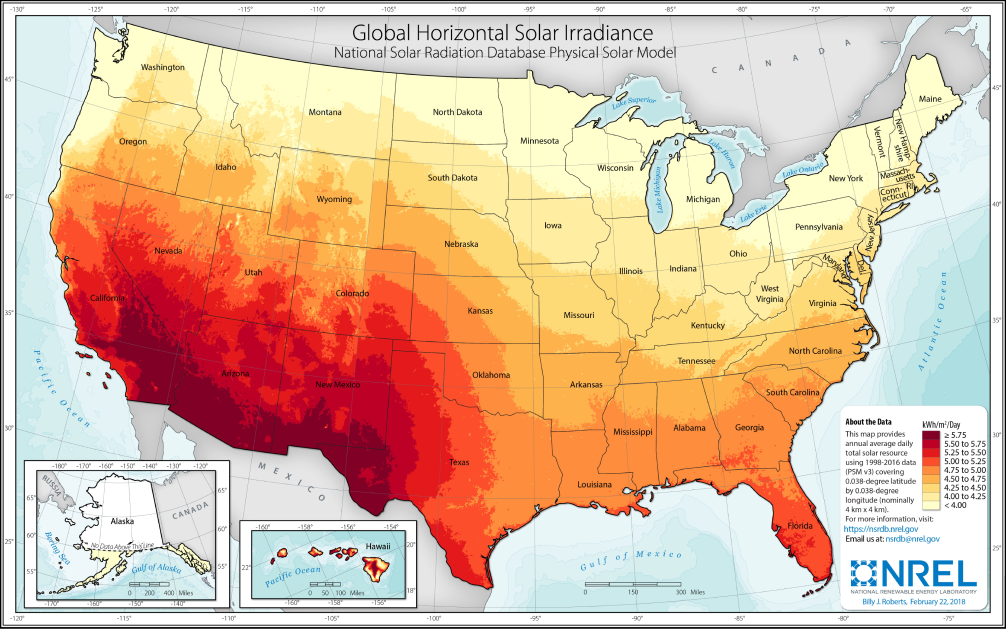
insolation map of the US[19]
At different parts of the world, we can optimize the amount of sunlight received by solar panels by tilting them in the direction of the sun, which is on average toward the equator.
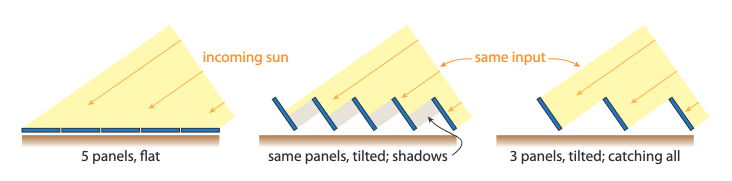
tilting solar panels toward the sun with separation is optimal[20]
In total, we get ~173,000 TW from the sun to our upper atmosphere, and ~123,000 TW makes it to the lower atmosphere.
With the current energy consumption of 18 TW, we would need to cover 0.4% of the land on earth with solar panels to meet our demands.
Solar is the only renewable energy source we've covered with the capacity to fully support current global energy needs and exponentially growing demands using existing technology.
Outlook on Renewables
We saw that many of the renewable methods are simply not abundant enough or too inefficient to cover global energy needs.
Two production methods in particular offer special promise for meeting our future energy needs: solar and nuclear.
Total global insolation is orders of magnitude more than enough to cover our current energy demands, and solar adoption is outpacing predictions.
In Part IV, we'll see how the grid demands a cheap, consistent, and reliable base source of energy. It currently depends on coal for this, we'll see that nuclear offers a great alternative to replace this.
In Part VII, we'll tie everything together by looking at how recent progress in solar and nuclear are changing our energy systems.