Energy storage systems allow us to transport energy across time and space by trapping it in forms that we can control.
There are 2 primary uses for energy storage: mobility and reserves.
Anything that needs to move around must carry it's own energy if it requires a continuous power source. This is why vehicles, personal computers, and mobile phones all have their own energy storage systems.
Additionally, whenever we want to preserve excess energy for later use, we need a storage system. Our body uses fat to store energy for the long-term, and the grid has it's own storage systems to maintain reserve capacity and smooth out imbalances.
The efficacy of an energy storage method is determined by:
- Charge Time - The time to store energy.
- Density - The amount of energy stored per unit volume or mass.
- Capacity - The amount of energy the storage system can hold.
- Power - The rate at which energy can be released by the system.
- Efficiency - The ratio of energy stored to energy released, affected by losses during storage and release.
- Lifetime - The number of charge-discharge cycles the energy storage system can undergo before it degrades.
Let's look at how modern energy storage systems work and evaluate them based on these constraints.
Fuel
We have already talked about the simplest form of energy storage: fuel.
A combustion power plant works by extracting the energy originally stored in it's fuel.
Fossil fuels like coal, oil, and natural gas store energy that originally came from sunlight.
They trap this energy in a form that's easy to transport and doesn't get released until we intentionally combust it.
Nuclear fuel is also a form of energy storage, where the storage system is the atomic nuclei themselves.
There is one difference between fuels and our modern energy storage systems.
Fuels release energy stored by nature, whereas modern storage systems release energy stored by humans.
We can see the relative energy density of different fuels and other energy storage systems below.
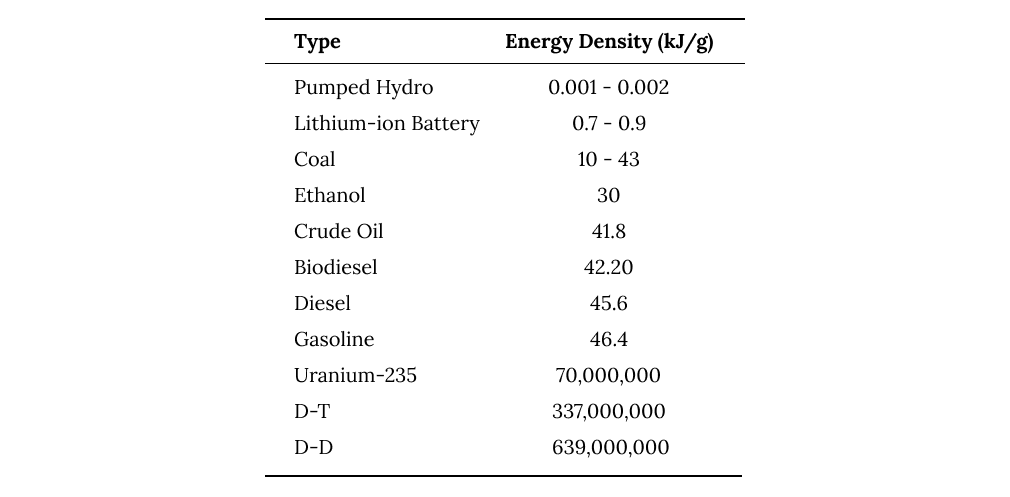
the energy density of fuels and other forms of energy storage
Pumped Hydro
Pumped hydroelectric provided the first form of grid energy storage.
In Part IV, we saw that ISOs maintain reserve production capacity in peaking plants to prevent outages and resolve last-minute grid imbalances.
Pumped hydro is a convenient energy source in both of these cases, since electricity generation can be turned on instantly.
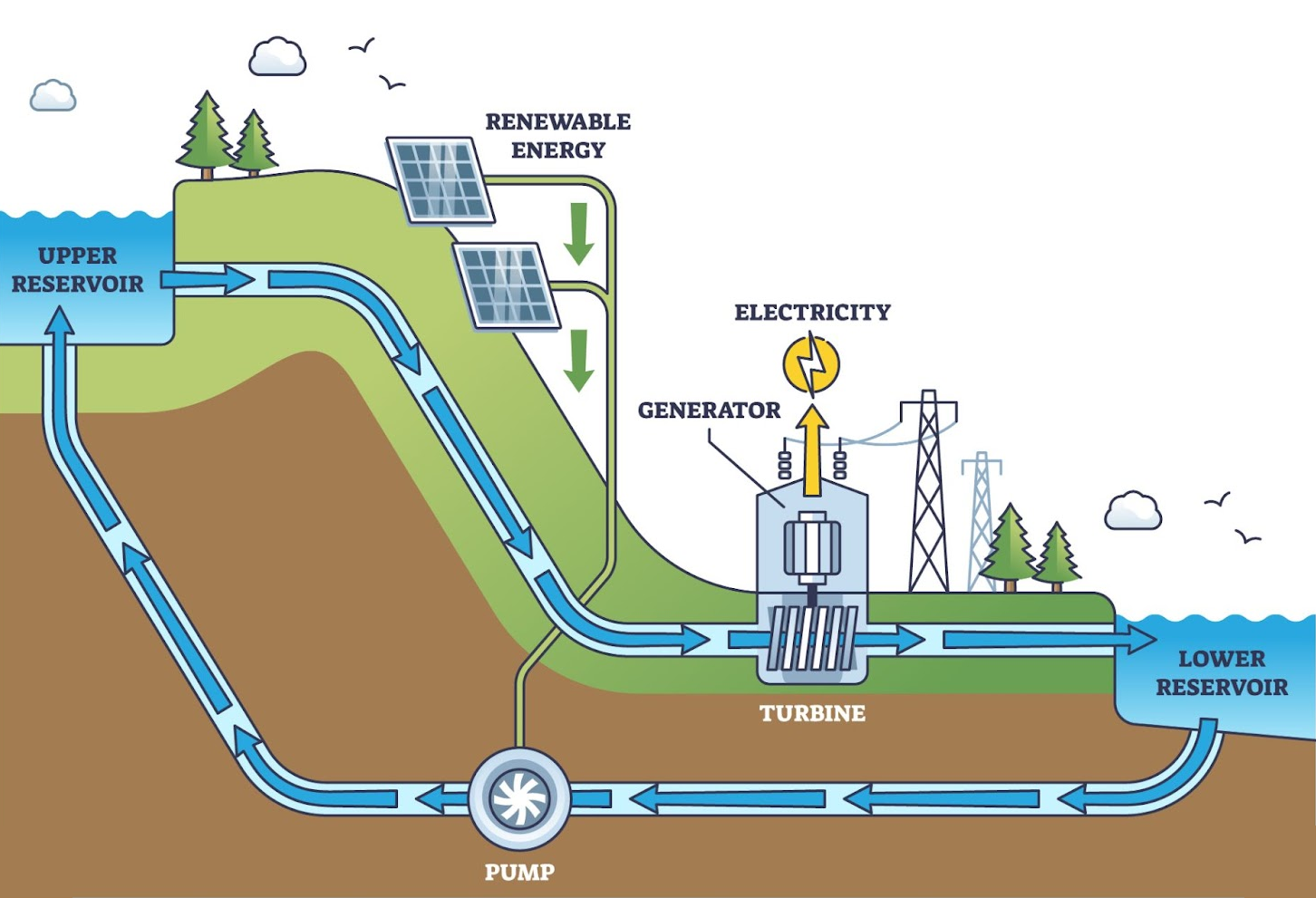
basic pumped hydro setup
Pumped hydro plants consume cheap electricity during the low-demand hours of the day to pump water from a low reservoir to a high reservoir.
This converts the electricity into energy stored in the increased gravitational potential energy of the water.
Then, they release this energy during peak demand when spot market electricity prices are high.
In this way, grid storage systems are a form of arbitrage between base and peak electricity prices throughout the day.
With enough grid storage deployment, electricity prices may converge such that the arbitrage spread narrows.
Aside: How energy dense is pumped hydro storage?
Pumped hydro systems have ~80% efficiency, meaning that 20% of the energy spent pumping the water is lost to friction.
We can use the formula for gravitational potential energy to calculate the energy density of this storage format:
Pumped hydro systems usually have a height difference of 200-800 m between their low and high reservoirs.
We'll assume an average height difference of around 400 m.
We can calculate that 1 kg of water in this setup has:
Only 80% of this energy is captured, or .
Finally, we can convert this to Wh/kg with the equivalence that that 1 Wh = 3600 J.
We see that 1 kg of water in this setup has 0.87 Wh/kg.
Batteries offer a much higher power density than this at ~30-150 Wh/kg.
In total, there's ~458 GWh of pumped hydro capacity in the world, making up ~90% of global grid storage. These storage systems are capable of a total power output of ~21 GW.
Pumped hydro systems also have a long lifetime. Though they take high upfront capital expenditure costing ~$1-2 billion to build, they can last many decades and recover their costs in this way.
Like we saw with hydroelectric generation capacity in Part III, opportunities to develop pumped hydro plants are rare.
Because of this, pumped hydro systems are unlikely to support the growing demands for grid storage brought by the increasing deployment of renewables.
Hydrogen
Hydrogen fuel has gotten a lot of attention over the past 2 decades.
It may seem like hydrogen should be framed as an energy production method since it can be reacted with to produce energy, similar to fossil fuels.
However, it's more accurately examined as an energy storage method.
The energy in hydrogen fuel must come from another energy source like natural gas or electricity.
In a sense, hydrogen is storing the energy from these sources to be later released.
Fuel Cells
The most basic unit used to extract energy from hydrogen fuel is the hydrogen fuel cell.
Hydrogen fuel cells consist of two metal plates called the anode and the cathode, separated by an electrolyte which allows ions to pass through it, but not electrons.
We'll see in the next section that this structure resembles that of a battery.
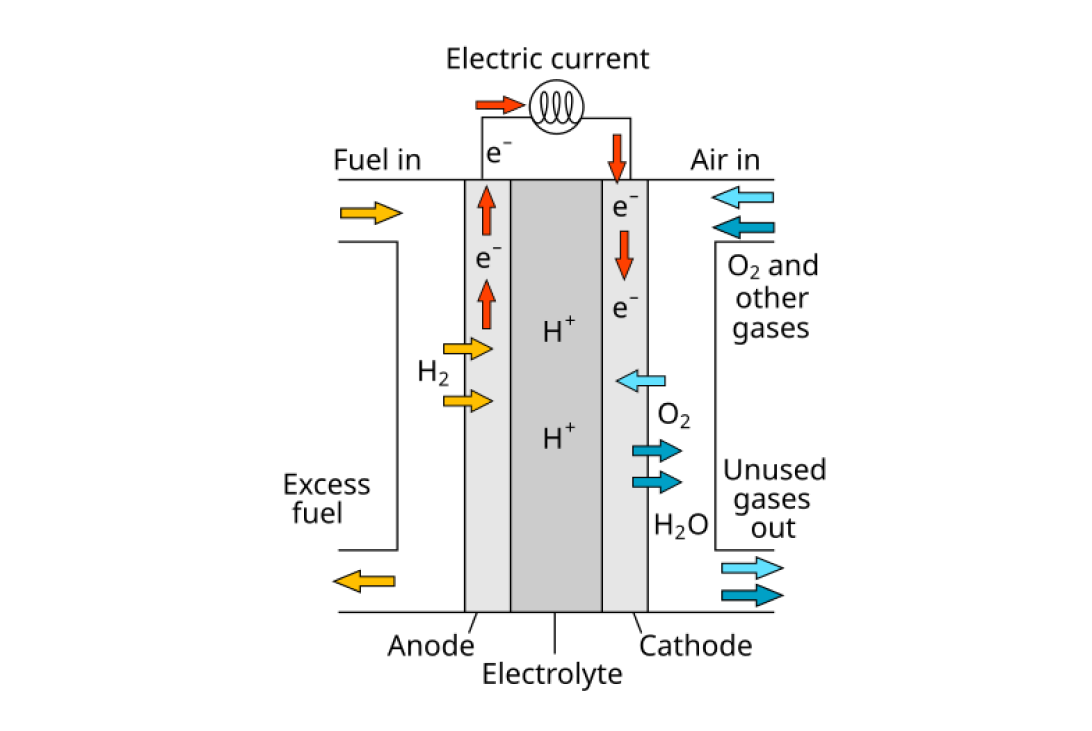
the function of a hydrogen fuel cell[1]
Hydrogen atoms are passed to the anode where a catalyst (often platinum) causes them to split into their individual proton and electron. The protons can then flow across the electrolyte to the cathode, where they join with oxygen on the cathode to form water.
Meanwhile, the electrons can't flow through the electrolyte, so they are instead pulled through a wire connecting the anode and the cathode, creating current and generating electricity.
We can get the oxygen for this process directly from the air, and the only waste produced is water.
Hydrogen Production & Storage
Hydrogen is the most abundant element in the universe, but it's so reactive that it always binds with other elements.
Lone hydrogen atoms are rarely found in nature.
In order to produce the hydrogen needed for fuel cells, we have to use energy to extract it from other sources.
Then, we have to store this hydrogen in isolated environments, so it can't recombine with other atoms again.
Aside: Hydrogen fuel types
Hydrogen fuel is categorized based on the energy sources that produced it.
The easiest way to produce hydrogen is through the combustion of natural gas with oxygen to produce hydrogen and carbon-dioxide.
This is known as gray hydrogen since it captures energy from burning fossil fuels.
Blue hydrogen is produced by systems that also combust natural gas but then capture the generated carbon-dioxide, reducing emissions.
The cleanest form of hydrogen, is produced by passing electricity through water, splitting the water molecules into hydrogen and oxygen.
This is known as electrolysis. When electrolysis uses electricity from renewables like wind and solar, the hydrogen it produces is known as green hydrogen.
There are a variety of other hydrogen types that exist today, though these 3 make up the most important categories.
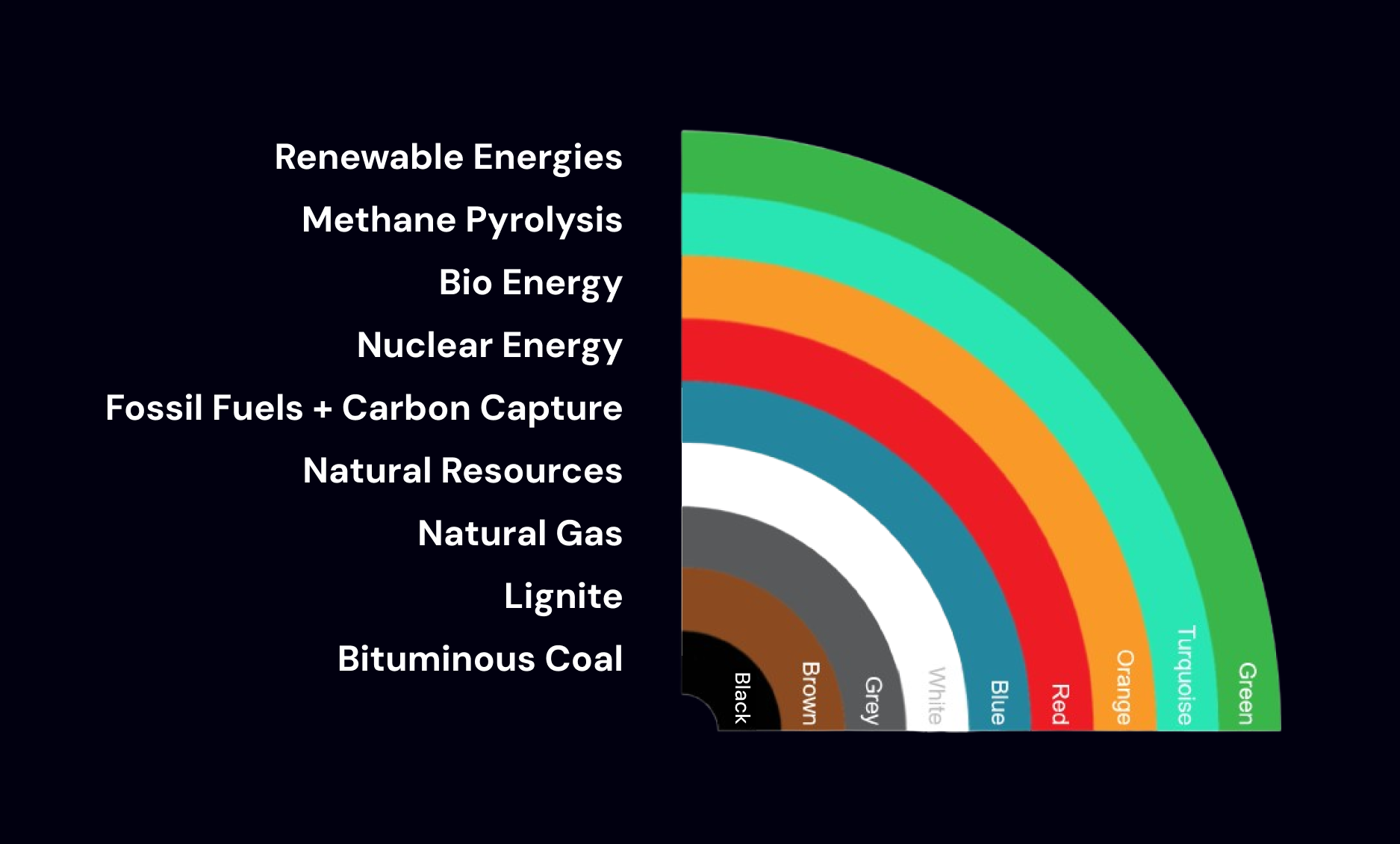
the different types of hydrogen based on energy source
Once produced, hydrogen gas has a high energy density by weight of ~120 kJ/g, compared with the 0.7-0.9 kJ/g energy density of most lithium-ion batteries.
However, hydrogen naturally has a very low energy density by volume of 0.01 MJ/L, compared with 0.9-2.5 MJ/L in batteries and 32 MJ/L in gasoline.
Hydrogen gas needs to be compressed to high pressures or cooled to -253 °C and liquefied in order to transport it effectively.
In this format, hydrogen can easily be stored in large tanks. This is more convenient than batteries which require more electrode material to increase capacity.
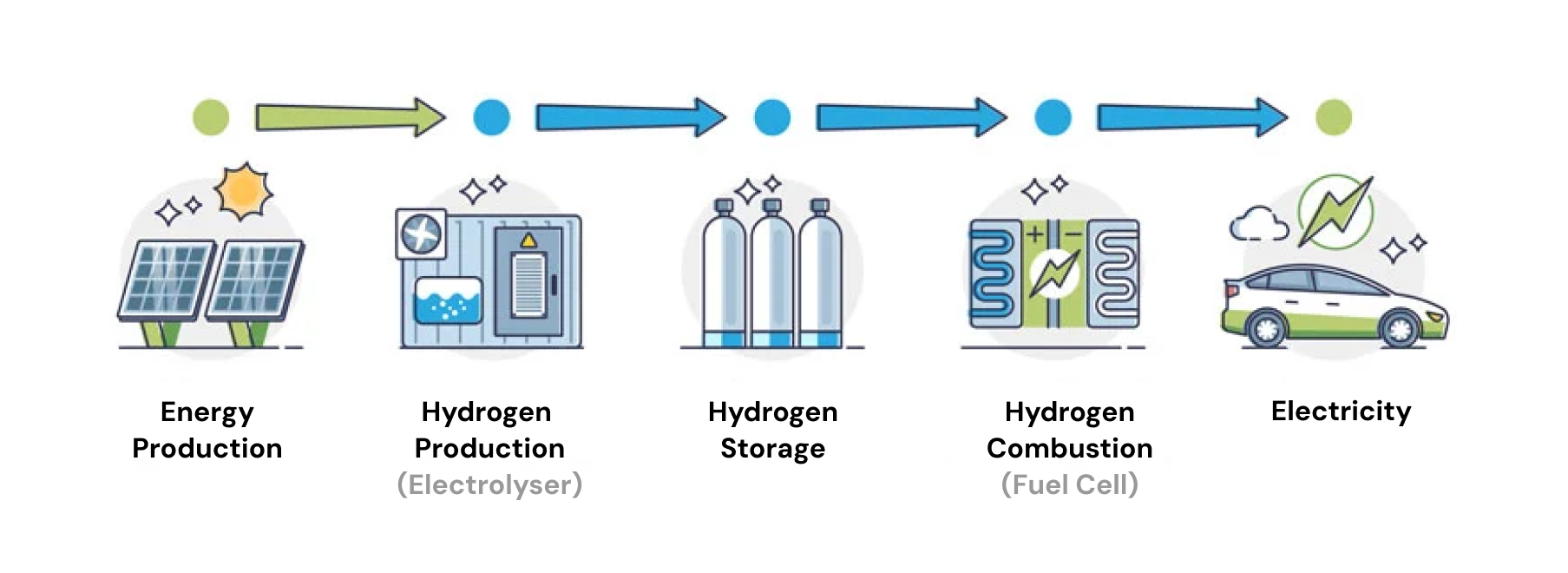
the full process of creating and using hydrogen for energy
With this context, we can see why the hydrogen economy seems promising.
Hydrogen's superior energy density and storage efficiency, combined with the prospect of clean hydrogen production, make it a very compelling alternative to replace the use of fossil fuels.
It seems especially useful for large-scale transportation like planes and ships where carrying large numbers of heavy batteries may be infeasible, and in heavy industry where the high energy density needs of industrial heating make batteries impractical.
Hydrogen also stores energy for longer than batteries, because batteries self-discharge, releasing energy even when they aren't being used.
Let's look at how the development of hydrogen technology has actually played out to see if it has fulfilled its potential.
Hydrogen Adoption
It has now been >20 years since George Bush announced his $1B Hydrogen Fuel Initiative to stimulate the US clean hydrogen industry in 2003.
In this time, hydrogen has far under-delivered on expectations.
Currently, hydrogen fuel is only used in large volumes for niche use cases like oil refining and fertilizer production.
<1% of global hydrogen fuel usage is consumed by newer use cases like transportation, making up a negligible portion of global use.
Despite tax credits and government funding, <0.7% of hydrogen usage comes from green and blue hydrogen, with the majority coming from fossil fuels.
Let's look at the factors that led to this outcome.
Hydrogen Realities
Hydrogen has several challenges that have prevented mass adoption.
First, the end-to-end process of producing, storing, and consuming hydrogen energy is inefficient.
The electrolysis process used to create green hydrogen has a 60-70% energy efficiency. Compressing, liquefying, and storing hydrogen introduces even more inefficiencies. Finally converting hydrogen back to electricity has a 40-60% efficiency.
The total round-trip efficiency of hydrogen is ~30-40%.
In comparison, batteries usually have a round-trip efficiency >80%.
Hydrogen production is also expensive.
For example, in California in 2014, the cost of a gallon of gasoline was ~$4. Meanwhile, the cost of an equivalent amount of hydrogen was $14.
Though this is an old example, it's illustrative of why hydrogen hasn't gained mass adoption.
Finally, hydrogen faces a massive infrastructure build-out challenge.
Building hydrogen refueling stations across the country would require the construction of nationwide hydrogen pipelines, resembling the scale of the oil and gas infrastructure build-out of the late 1800s and 1900s (though the oil and gas build-out was funded by free market incentives rather than government subsidies).
Meanwhile, batteries and electrical vehicles had a much easier time with this challenge given the widespread distribution of existing electrical grids.
This made it much more feasible for companies like Tesla to build charging network across the country.
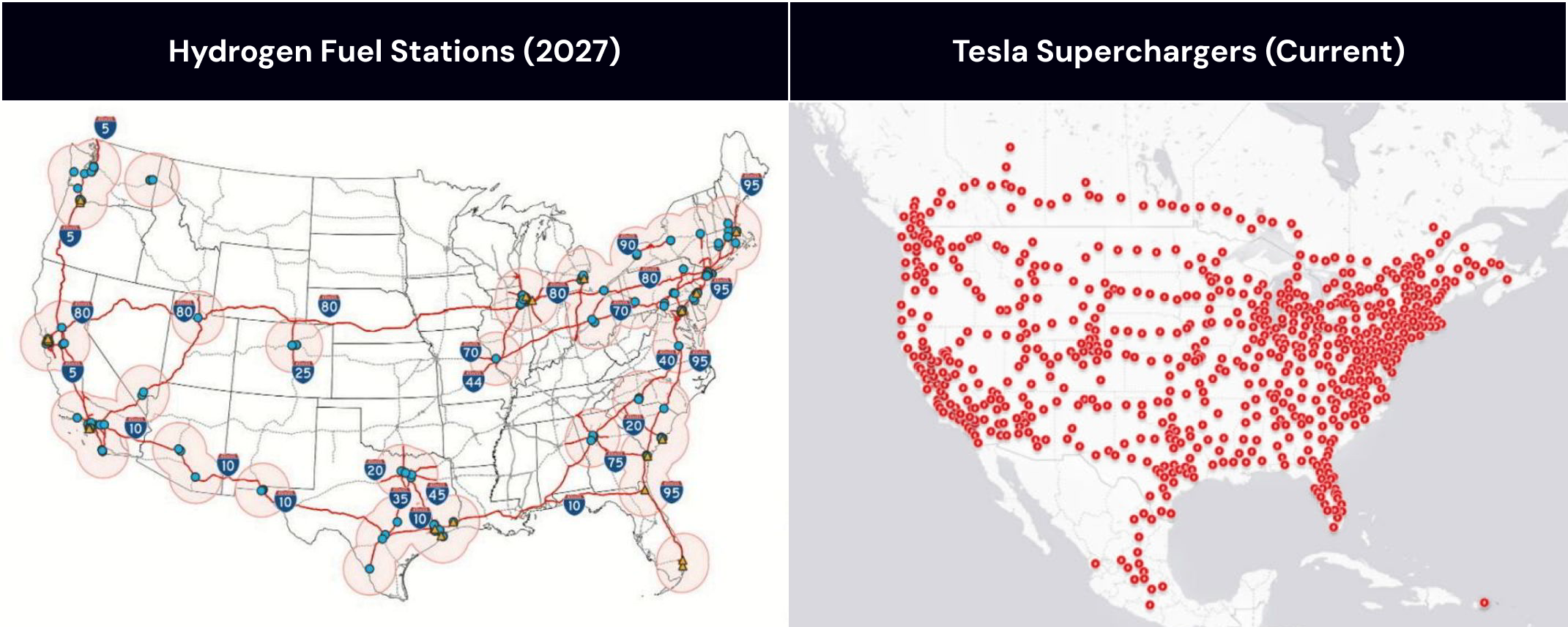
hydrogen fuel stations (2027 forecast) vs. Tesla supercharger network
The present realities of the hydrogen energy look far less impressive than the expectations created by hype and narratives.
However, it would be premature to say that the hydrogen economy is bound to fail.
Hydrogen technology is still early in its development curve, with recent improvements in electrolyzer processes improving hydrogen fuel efficiencies and costs.
Funding continues to flow in from governments across the world via tariffs and stimulus.
The Biden-Harris administration announced a $7 billion stimulus for the build-out of clean hydrogen fuel hubs across the US in 2023.
The EU, China, Japan, and many other governments have also included hydrogen production in their energy development plans.
Regardless, it's unclear how much farther the hydrogen economy must develop before it's ready for mass adoption, and whether it can get to this point supported primarily by government stimulus.
In practice, every technology that has gotten widespread adoption did so by first becoming economically viable for a small market, which it could use to drive down costs and further innovate.
This is how the semiconductor industry developed, and is the reason why the adoption of solar, wind, batteries, and electric vehicles has taken off in the past decade (we'll look more into some of these trends in Part VII).
In contrast, the main driver for the continued development of hydrogen technology is that it may be the only way to shift heavy industry to clean energy.
However, hydrogen must be cheaper, not just cleaner, in order to actually gain market share. Currently, hydrogen does not meet this requirement.
With no economically viable market wedge yet, it's unclear how well the hydrogen economy will fare as competing technologies far outpace its rate of innovation.
Batteries
Batteries are the fastest growing energy storage technology.
They've already enabled the adoption of mobile phones and electric vehicles over the past 2 decades, and are now positioned to augment the grid.
Let's look at how batteries work and why they have been gaining market share much faster than other storage technologies.
Structure
The simplest unit of a battery is an individual cell.
Each battery cell has two metal electrodes of opposite charge, separated by a material that prevents the flow of electrons between the electrodes.
We call the negatively charged electrode the anode, the positively charged electrode the cathode, and the separator material the electrolyte.
The battery cell can store energy in the form of a charge difference between the two electrodes. This is known as electrochemical potential.
When a wire is connected between the two electrodes, electrons are attracted from the positive electrode to the negative electrode through the wire.
This resolves the charge imbalance between the electrodes and generates current in the process.
The specific properties of a battery depend on the materials selected for each of its parts.
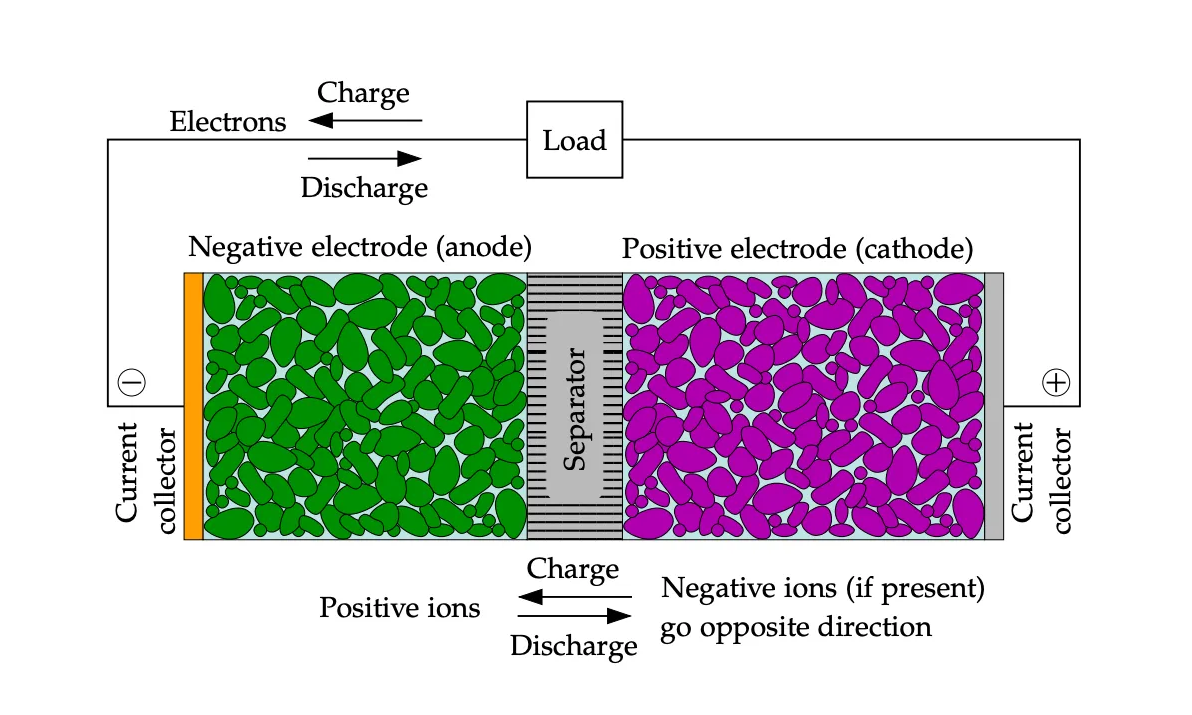
structure of a single battery cell[2]
The anode is usually made of a metal or alloy (like zinc or lead) with a tendency to lose electrons.
The cathode is usually made of a metallic oxide (like manganese-dioxide) with a tendency to gain electrons.
Due to the anode's tendency to lose electrons, and the cathode's tendency to gain electrons, an electric potential difference (voltage) forms between the anode and cathode.
We can see some example voltages based on different battery electrode compositions below.
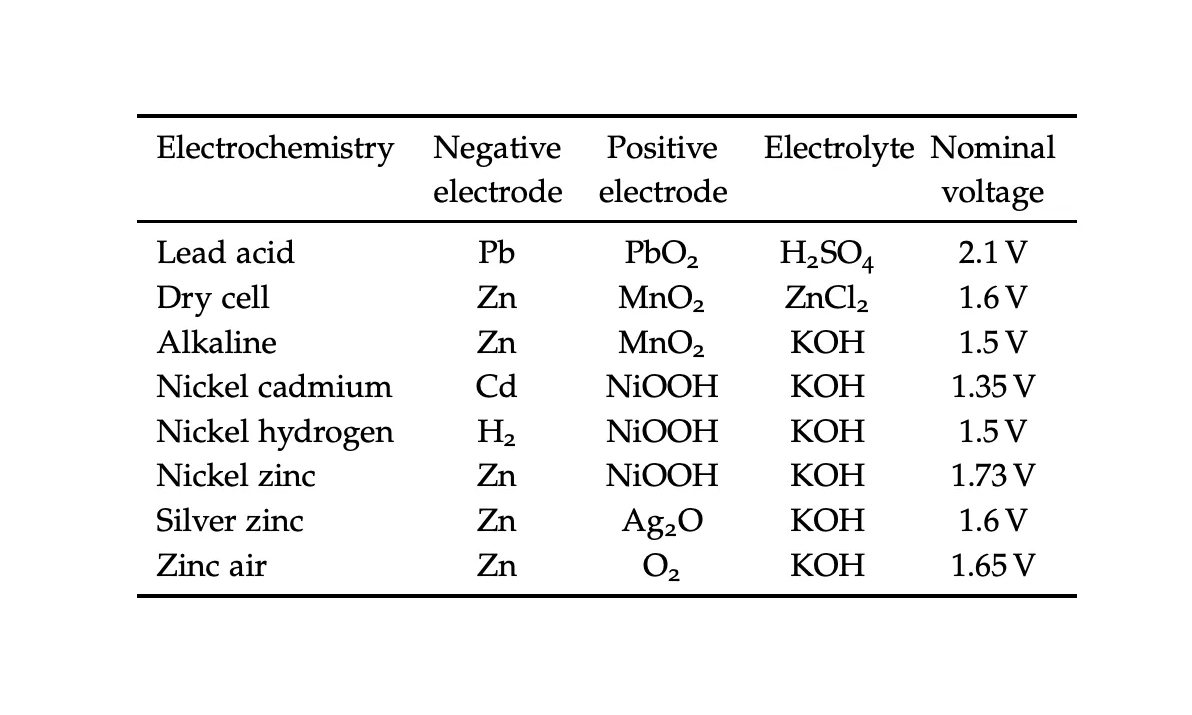
potential differences created by different anode and cathode materials[2]
This voltage across the electrodes is what drives electrons from the anode to the cathode during discharge.
Given , we know that batteries with higher voltages due to the materials in their electrodes will supply more total power.
In addition to preventing electron flow between the electrodes, the electrolyte in most batteries directly reacts with the metals in the electrode.
During discharge, the ions in the electrolyte react with the metals in the anode.
These reactions create metal-oxides, and electrons are released in the process. This is what actually frees electrons to generate current.
After discharging, a battery can be recharged by applying a voltage to pump electrons back from the cathode to the anode and reverse the chemical reactions in both electrodes.
This recreates the voltage difference across the battery terminals by expending energy, effectively storing that energy in the battery.
The small cylindrical battery format most people are familiar with is known as a battery roll.
This device contains many sheets of joined cathodes, electrolytes, and anodes rolled into a single capsule with positive and negative terminals at either end contacting the respective electrodes.
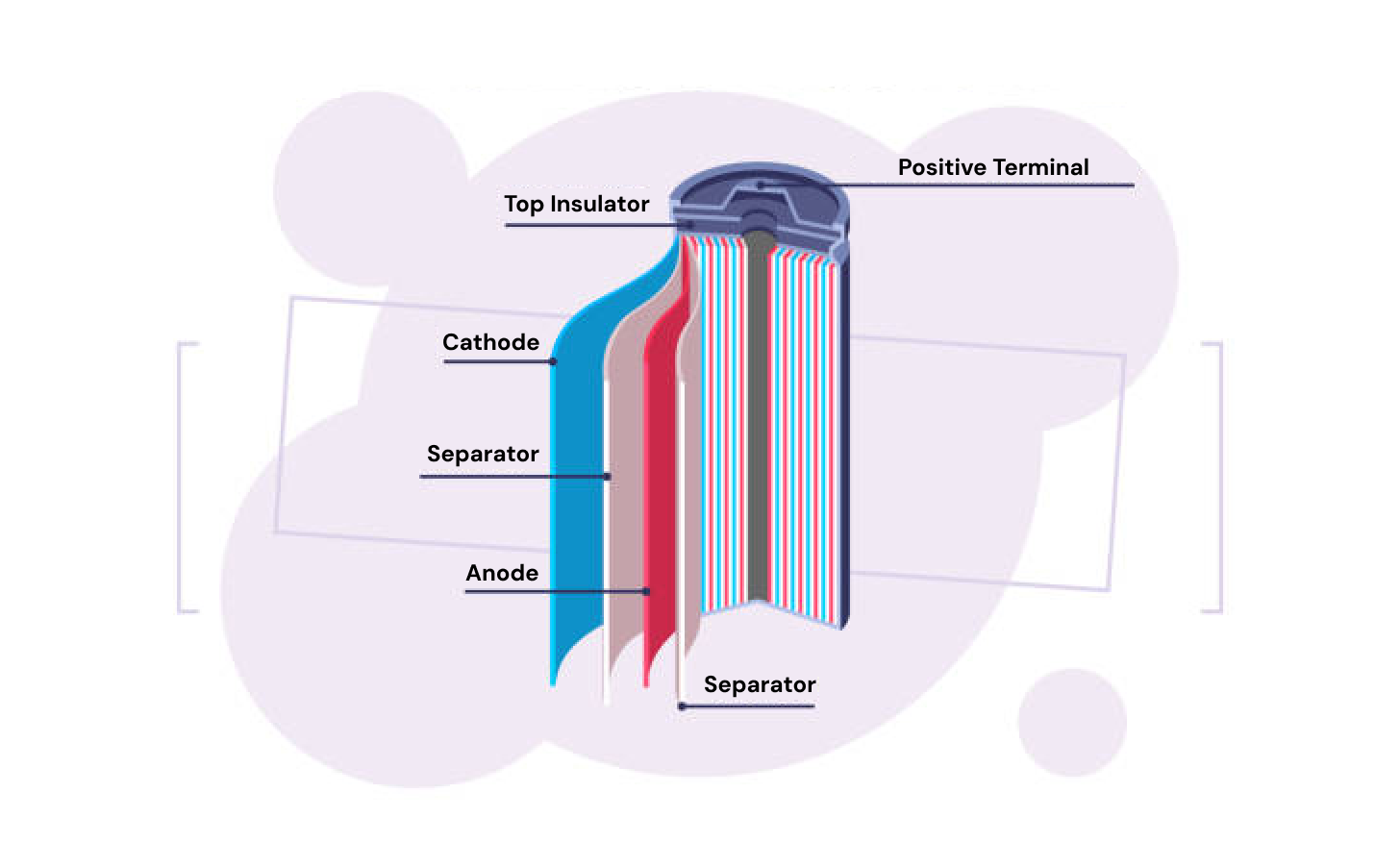
an individual battery roll contains many rolled up sheets of electrodes and electrolytes
Aside: Early battery chemistries
Lead Acid
The lead-acid battery was the first commercially viable rechargeable battery, invented by Gaston Planté in 1859.
It uses lead () in the anode and lead-dioxide () in the cathode, which creates a voltage of 2.1 V across the electrodes.
These metals react with sulfate ions () in the electrolyte during charge and discharge to store and gain electrons.
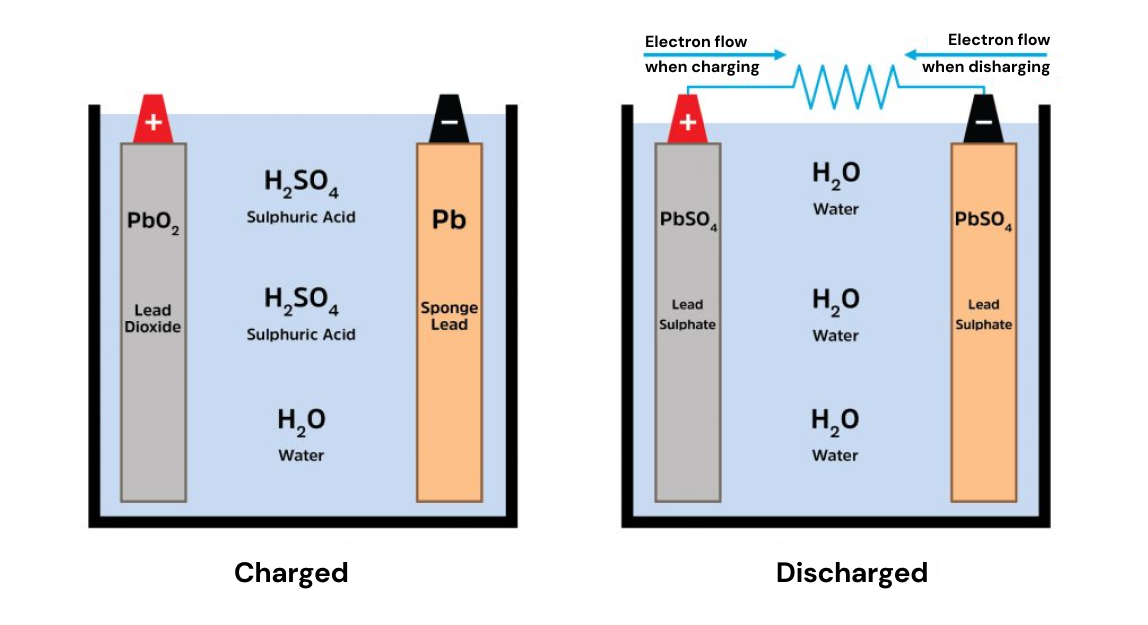
composition of a lead acid battery during charge and discharge[3]
These batteries gained widespread adoption in car starters in the early 1900s due to their reusability and the abundance of lead, making these batteries relatively cheap and available.
Due to the heavy metals present in electrode reactions, they had a relatively low energy density at ~30-40 Wh/kg.
This was not an issue for early automobiles which were already heavy and had short ranges around 40-50 miles.
However, these batteries were impractical for mobile applications due to their weight.
Lead acid batteries degrade over time as metal reactions breakdown the electrodes.
Because of this, they have a maximum lifetime of ~300-500 charge-discharge cycles.
Nickel Cadmium
The nickel-cadmium () battery gained widespread adoption in early electronics in the 1950s.
These batteries used cadmium () in the anode and nickel oxyhydroxide () in the cathode.
Both of these metals are lighter than lead, leading to a nickel-cadmium battery energy density of ~45-80 Wh/kg vs. lead-acid's 30-40 Wh/kg.
Because of this, it was the perfect battery option for early mobile electronic devices like power tools, portable radios, and early laptops.
However, these batteries have an issue known as the memory effect where incomplete discharges followed by recharges trap some cadmium permanently in crystalline structures, reducing the overall capacity of the battery.
Though longer lasting than lead-acid batteries, these batteries still had lifetimes of ~500-1500 cycles, and their energy density was not good enough for small electronics.
Lithium-ion
Lithium-ion cells have made it possible to use batteries for previously infeasible use cases like small portable electronics and electric vehicles.
There are 2 primary differences between lithium-ion batteries and other chemistries that account for their superiority.
Lithium
First, lithium-ion batteries use lithium ions that can travel through the electrolyte as the primary charge carriers rather than the stationary metals in the electrode.
This removes the need for heavy electrode materials like lead or cadmium, which were essential in earlier batteries to carry electrochemical potentials.
Because of this, lithium batteries use much lighter electrode materials like graphite that reduce the overall weight of the battery.
Lithium itself is light and very electronegative.
In fact, lithium is the 3rd lightest element in existence.
Since it only has 1 electron in its valence shell, it's also highly reactive, wanting to give up its electron easily.
Because of this, lithium-ion batteries have a much higher voltage than other chemistries. This allows much fewer battery cells to serve the same power needs, leading to lighter battery systems.
Intercalation
Second, lithium ions don't chemically react with the electrode materials during charge and discharge.
This contrasts earlier battery chemistries where ions in the electrolyte have to react with metals in the electrodes for current to flow.
Instead of reacting, lithium ions are stored in the spaces between atoms in the electrodes. This is known as intercalation.
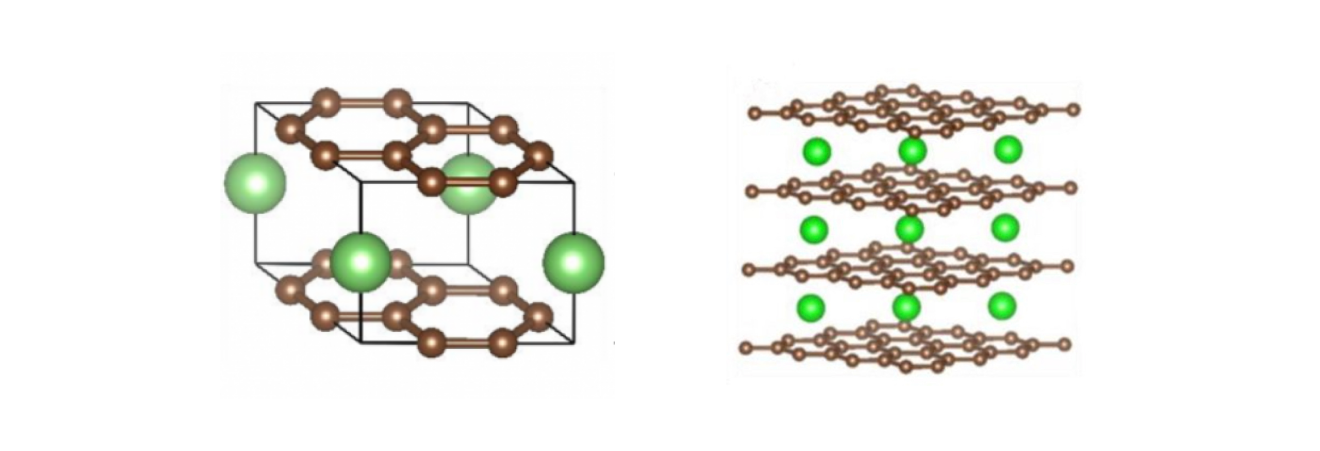
lithium atoms (green) intercalating between sheets of graphite (brown carbon atoms)
This enables faster ion movement since charges are free to move without reacting, leading to faster charge and discharge times.
It also reduces the degradation of electrodes since there aren't constant chemical reactions that destroy the electrode metals.
Finally, the physical arrangement of intercalation allows more lithium ions to fit within the electrode structure, leading to higher energy densities.
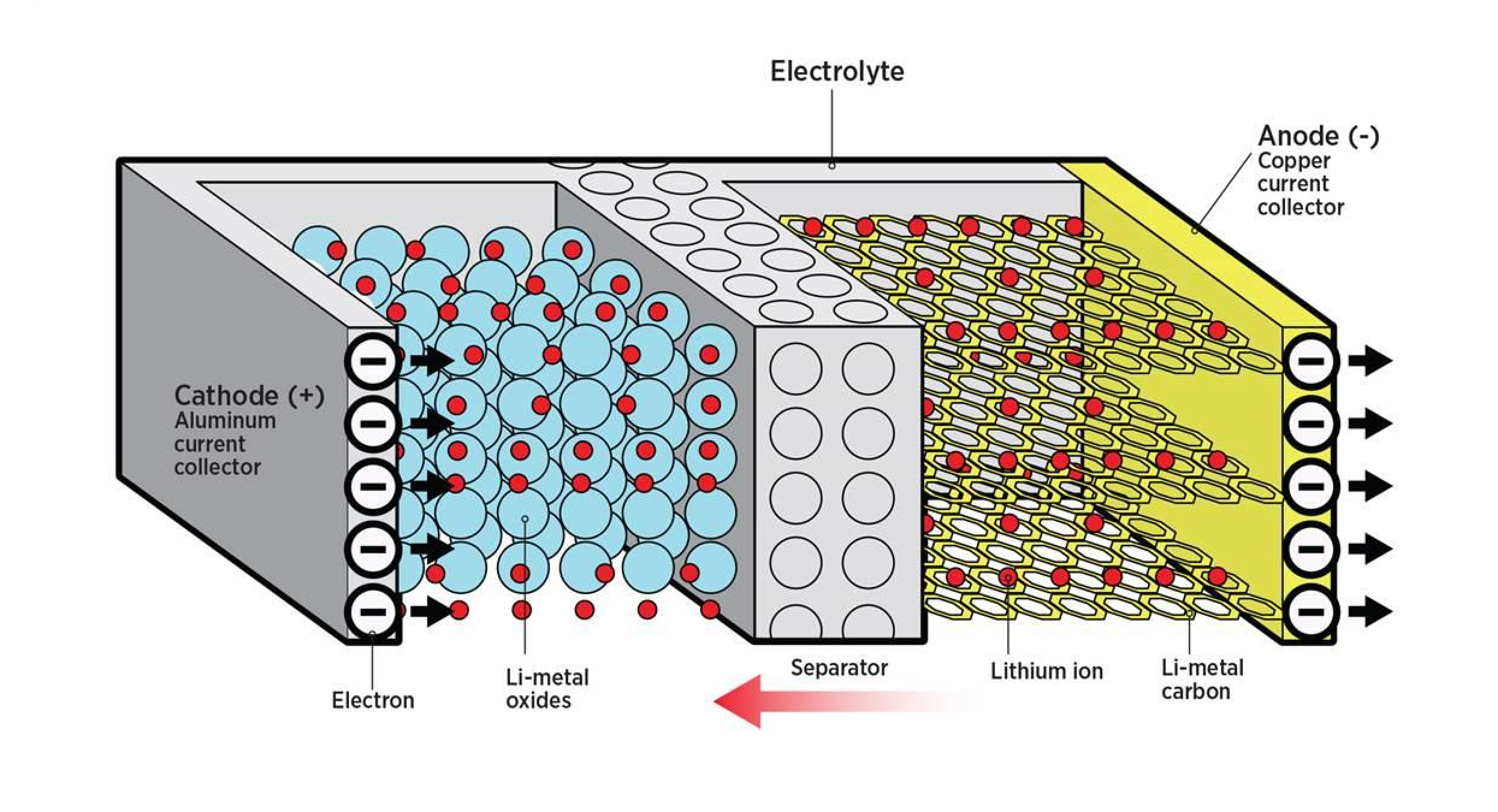
intercalation visualized in the electrodes of a lithium-ion battery
Aside: The solid electrolyte interface
The original lithium-ion battery chemistry, invented by Sony in 1991, used graphite in the anode and lithium-cobalt-oxide (LCO) in the cathode.
During charge, some of the lithium ions coated with the electrolyte unexpectedly reacted with graphite in the cathode, forming a solid electrolyte interface (SEI) between the graphite and the electrolyte that consumed ~5% of the battery's total lithium.
It turned out that SEI prevented electrons from touching the electrolyte.
Electrons typically degrade the electrolyte over time, so the SEI actually increased the lifespan of the battery.
Now, battery engineers work to optimize the chemistry of the SEI to improve lithium-ion battery properties and maximize lifetimes.
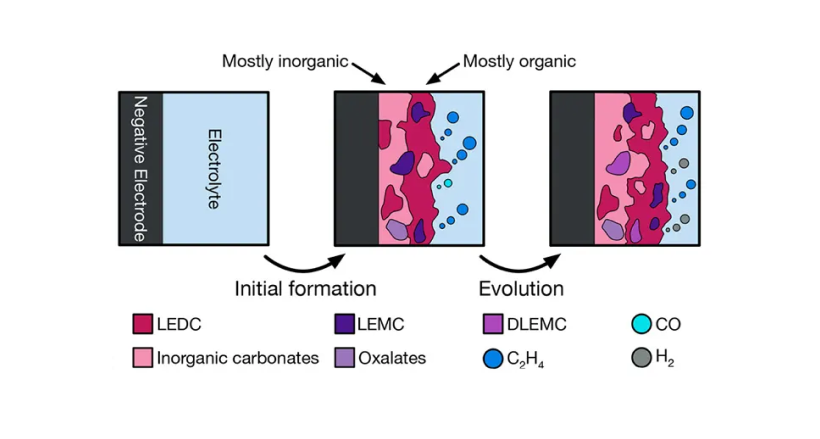
formation of the SEI[4]
Advantages & Challenges
Altogether, these factors contribute to make lithium-ion batteries:
- Lighter and more energy dense, with energy densities around 150-250 Wh/kg, compared with 45-80 Wh/kg in NiCd and 30-40 Wh/kg in lead-acid.
- Higher voltage, with ~3.6-3.7 V per cell, compared with 2.1 V in lead acid, and 1.2 V in NiCd.
- Faster to charge and discharge with charge times around 1-4 hours compared with 8-16 hours in other batteries.
- Longer lasting, with different lithium-ion chemistries lasting 2-3x longer than other battery types.
- Lower self-discharge. Nickel cadmium and other cells often lose 1-5% charge every day even when not connected, whereas lithium-ion cells can retain their charge after months. This is critical for any end-uses that require longer-term storage.
Lithium-ion batteries are far superior to other chemistries in these dimensions, but they come with some challenges.
Most notably, lithium-ion batteries can be damaged by overheating. Excess heat can break down the electrolyte and cathode, leading to a chain of chemical reactions that produces more heat known as thermal-runaway.
They're also susceptible to being overcharged, which can cause lithium that can't intercalate to buildup as lithium metal plating on the anode, reducing battery capacity and increasing the risk of short circuits. Overcharging also runs the risk of decomposing the electrolyte.
Modern battery management systems used in electric vehicles and grids have robust thermal and charge management systems to mitigate these issues.
Battery Engineering & Adoption
The widespread adoption of lithium-ion batteries over the past 2 decades has been a result of successful battery engineering that has created cheaper, safer, and more energy dense battery chemistries.
All these chemistries have different properties and trade-offs.
Battery engineering is about selecting the materials for the positive and negative electrode and electrolyte to maximize power and capacity, while maintaining trade-offs in lifetime and safety.
The electrode materials selected determine:
- The voltage across the battery, based on the electric potential difference between the electrodes.
- The resistance of the battery, based on how easy it is for lithium to intercalate and de-intercalate from electrode crystal structures.
- The current of the battery, determined by resistance and voltage.
- The power output of the battery, a function of the voltage and the current.
- The capacity of the battery, based on how densely the lithium can pack into electrodes.
Aside: Lithium-ion battery chemistries
Let's briefly look at how materials choices in the most popular lithium-ion battery chemistries lead to different battery properties.
Lithium titatine oxide (LTO) batteries use lithium-titanite in their anodes instead of graphite.
Lithium-ions are able to intercalate much more quickly in lithium-titanate than in graphite, which makes LTO batteries much faster for charging and discharging.
This can be useful in heavy-use applications like electric bikes that are charged and discharged every day.
However, lithium-titanate also has a higher electrochemical potential, bringing it closer to the potential of the cathode.
As a result, the battery has a lower voltage across the electrodes. LTO batteries typically have a voltage ~2.2-2.4 V compared with the 3.6-3.7 V in standard LCO batteries.
There's also interest in developing sillicon-based anodes for lithium-ion batteries.
Lithium ions intercalate much more densely in silicon than in graphite, which would lead to a >5x improvement in energy density.
However, silicon also expands more when lithium intercalates, leading to more breakdown which could decrease the total lifetime of the battery.
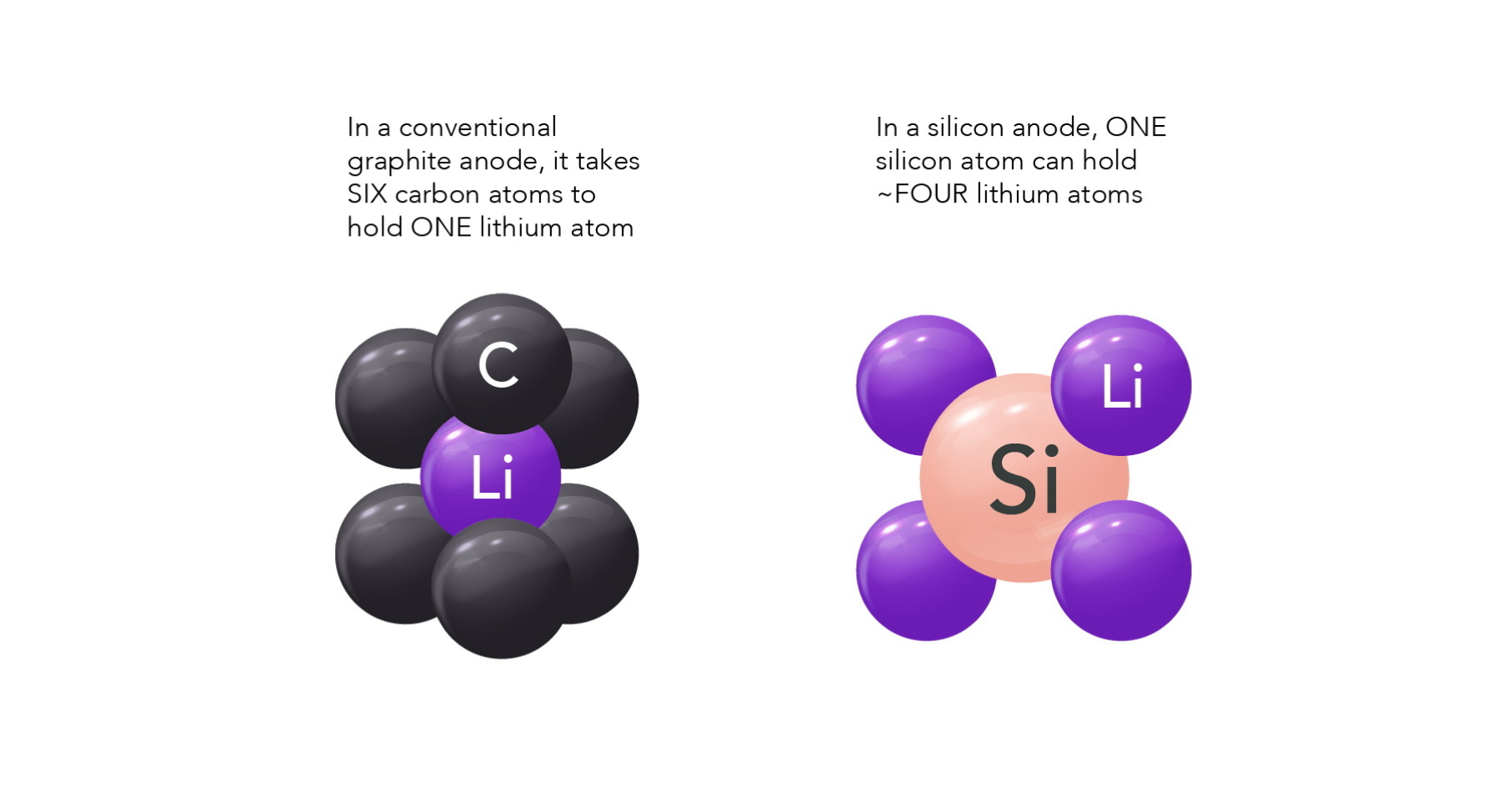
lithium intercalates more densely in silicon than in graphite
Cost is also a big concern for battery usage, especially at the scale of grid storage.
Battery costs are primarily driven by the materials that make up their electrodes
LCO batteries are expensive because cobalt is rare, so cheaper alternatives like nickel mangagnese cobalt oxide (NMC) and lithium nickel cobalt aluminum oxide (NCA) electrodes are often used, which still use cobalt, but in much smaller proportions.
Tesla primarily uses NCA batteries in its long range vehicles like the Model S and X due to its cost effectiveness and high energy density.
The finally chemistry worth mentioning is lithium iron phosphate (LFP) batteries, which are cheap and non-toxic due to their lack of cadmium use.
However, lithium in this electrode material intercalates in linear channels, slowing down ion diffusion and increasing resistance.
Tesla primarily uses LFP batteries for their shorter-range vehicles like the Model 3 and Y due to low costs, longer life cycles, and better safety.
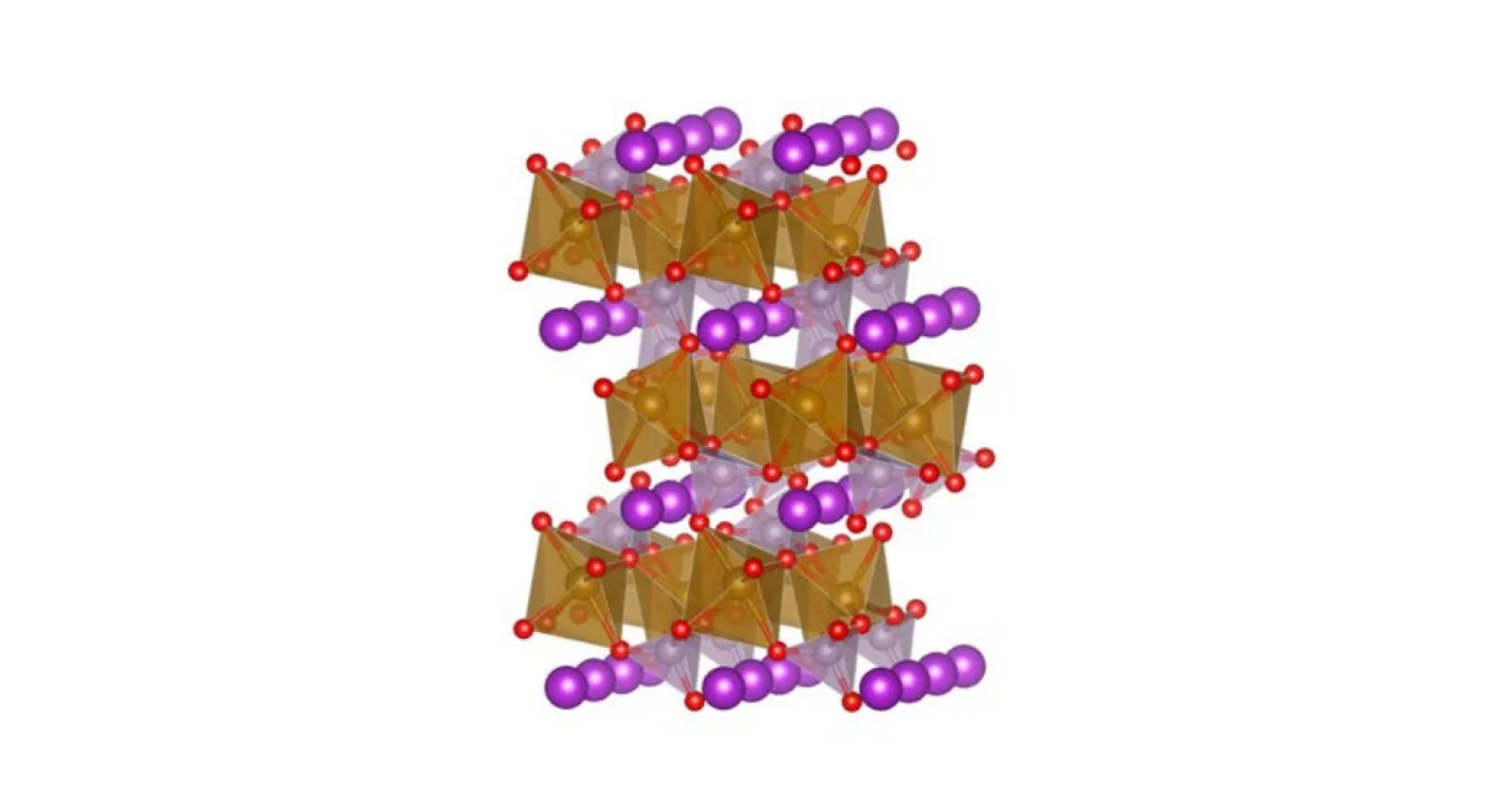
linear channels for lithium (purple spheres) intercalation in LFP batteries[5]
The lithium-ion battery industry has periodically used funding from one application to develop new chemistries with better power outputs, capacities, and energy densities, making batteries viable for new applications.
Lithium-ion batteries were initially used for small personal computers and mobile electronics.
As revenues from these sources drove innovation, they became suitable enough to be used in electric vehicles, which has now become their largest use.
In electric vehicle battery packs, multiple battery cells are combined in series and parallel. This increases voltage and current of the battery pack to meet the vehicle's power needs.
These battery cells are then combined with thermal and electrical control systems to make them safe.
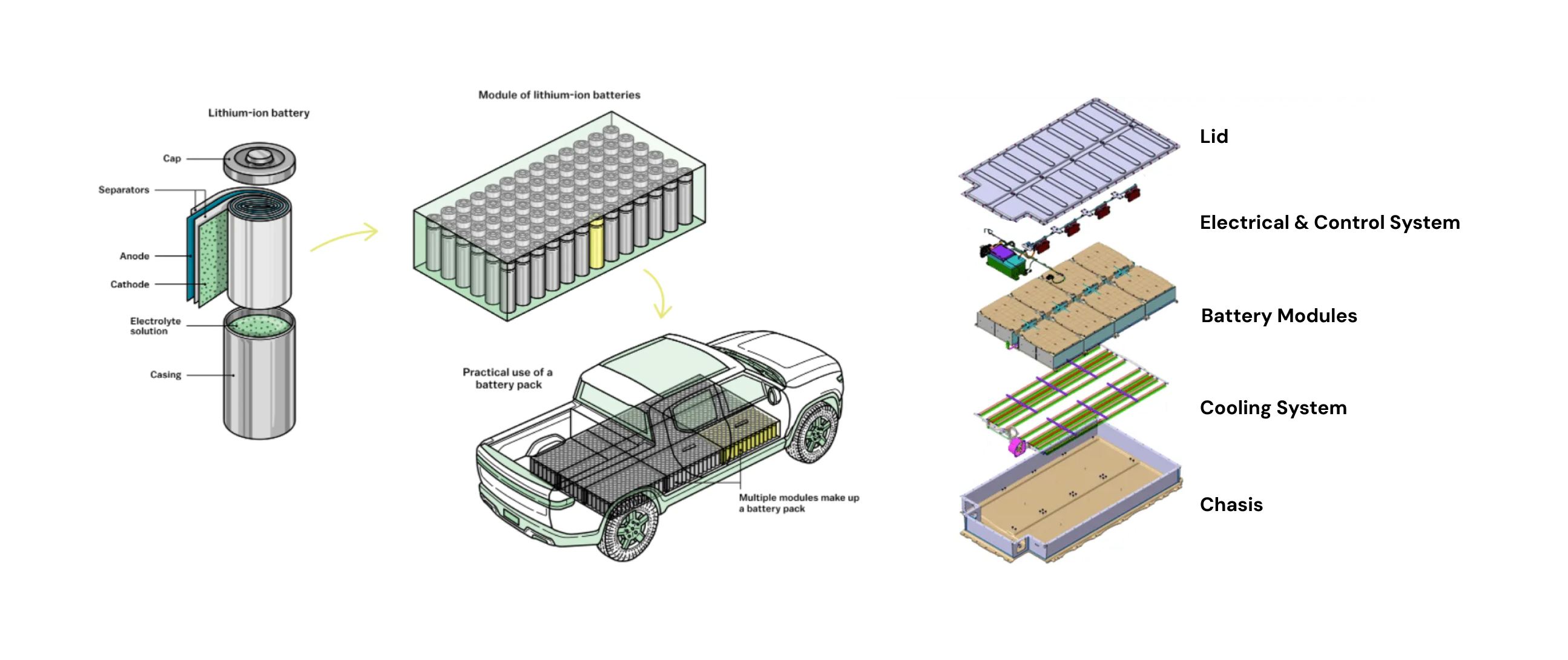
structure of an electric vehicle battery pack
The development of batteries for electric vehicles has brought the technology to the point where it can be used in battery energy storage systems (BESS) for grid storage.
BESSs use inverter-based power conversion systems (PCS) and medium-voltage transformers (MVTs) to convert the output of large blocks of batteries to high voltage AC currents that can be deployed to the grid.
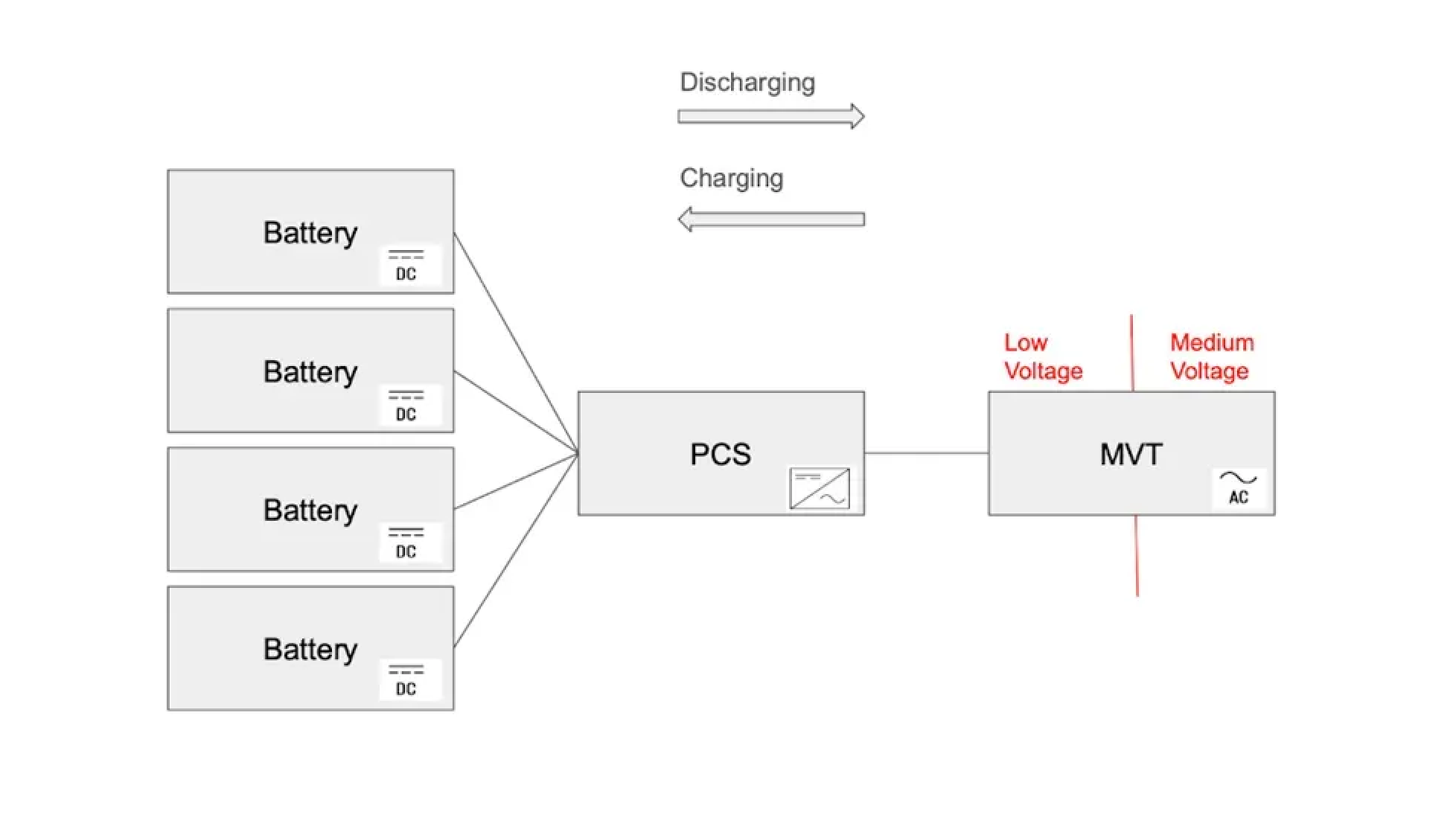
basic battery energy storage system setup[6]
They also use monitoring tools and software to ensure that batteries stay within thermal limits and that charging gets distributed evenly across cells.
BESSs are sold in 20-ft containers with ~20 MWh capacity that are now being developed by Tesla, BYD, CATL, and many others. In Part VII, we'll see that the number of BESS deployments on the grid is growing quickly.
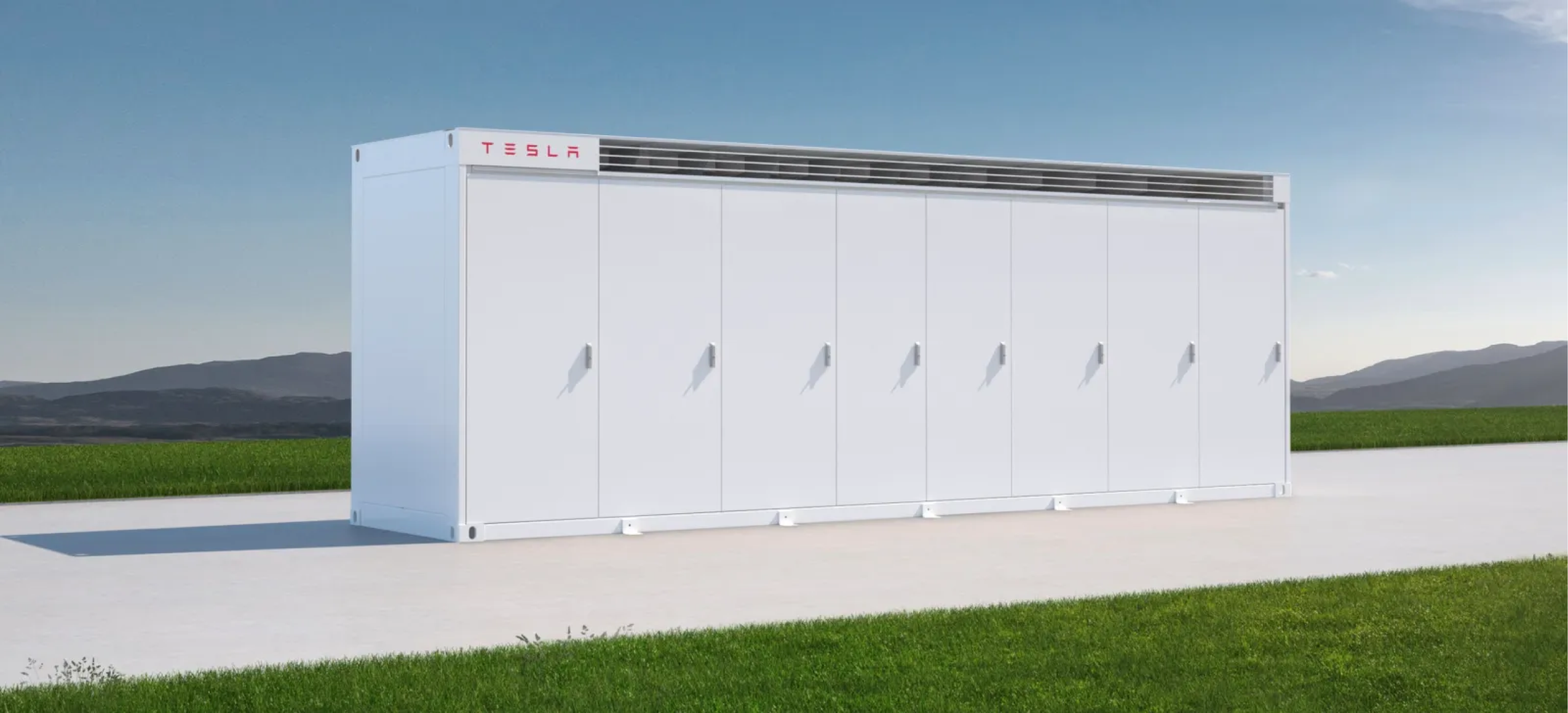
Tesla's Megapack BESS
Outlook on Batteries
Lithium-ion batteries are cheaper, charge faster, last longer, and have higher energy densities than previous chemistries.
The widespread adoption of lithium-ion batteries in different industries has consistently unlocked new use cases for batteries to disrupt.
They have already successfully transformed energy storage in personal computation and transportation, and are now primed to change grid storage.
We'll discuss this phenomenon in more detail in Part VII.